Stereotactic radiosurgery with charged-particle beams: technique and clinical experience
Introduction
The application of charged-particle radiation to stereotactic radiosurgery has been the subject of biomedical research and clinical development for almost 60 years (1,2). In 1946, Wilson (3) first proposed the clinical use of charged-particle beams because of their unique physical properties. After completion of the 184-inch synchrocyclotron at the University of California at Berkeley, Lawrence Berkeley National Laboratory (LBNL) in 1947 (4), Tobias and colleagues (5) began studies of the radiobiological effects of narrow beams of protons, deuterons and helium ions. Particular attention was paid to the reaction of normal brain tissues to charged-particle irradiation for subsequent clinical application to radiosurgery (6). The range of medical applications was constrained initially by the limitations of available neuroradiological techniques for stereotactic localization, image correlation, and treatment planning (2). Early clinical trials were therefore restricted to selective destruction of small, well-defined target volumes that could be localized accurately by existing neuroradiological procedures. Stereotactic irradiation of the pituitary gland was among the earliest applications, because localization of the sella turcica bone structure at the skull base could be accomplished reliably with plain radiographs.
The very first human treatments with charged-particle beams in 1954 were delivered with a radiosurgical technique: defined as carefully delineating intracranial target volumes, stereotactically localizing these volumes within a rigidly immobilized patient, and hitting these targets with millimeter accuracy using isocentrically focused radiation beams delivered with high fractional doses. The initial clinical trials used high doses of protons (and then helium ions) to ablate the pituitary gland for palliative treatment of metastatic breast cancer by means of pituitary-hormone suppression (7,8). Charged-particle radiosurgery was then applied to the pituitary-ablation treatment of proliferative diabetic retinopathy (9), and to the treatment of pituitary adenomas (10,11). With the development of improved techniques of stereotaxis and cerebral angiography, charged-particle radiosurgery was applied to the treatment of arteriovenous malformations (AVMs) (12-14).
In the mid-1970s, the advent and rapid evolution of high-resolution computed X-ray tomography (CT) enabled much improved calculation of the three-dimensional (3D)-depth-dose distribution of charged particle beams (15). When these dose-localization improvements were coupled with the higher-quality anatomic definition of magnetic resonance imaging (MRI) (16), it became possible to extend the use of charged-particle beams to a much wider variety of life-threatening disorders, including many types of cancer throughout the body, and to conventionally fractionated radiation therapy (RT). Some 100,000 patients worldwide have now been treated with charged-particle beams at more than 30 institutions (17). The experience with standard fractionation protocols of charged-particle therapy will be discussed elsewhere in this edition of Translational Cancer Research. This review will be limited to describing the worldwide clinical experience with charged-particle radiosurgery in the treatment of more than 15,000 intracranial patients and in more than 12,000 ocular patients.
Physical properties of charged-particle beams
Charged-particle beams of proton mass or greater (e.g., helium, carbon, and neon ions) manifest unique physical properties, first observed by Bragg in 1904 (18), that can be used to place a high dose of radiation preferentially within the boundaries of a deeply located intracranial target volume (Figure 1) (2). These include: (I) a well-defined, energy-specific range that can be modulated so that the beam stops at the distal edge of the target, resulting in little or no dose beyond the target; (II) an initial region of low dose (the plateau ionization region) as the beam penetrates through matter, which is followed deep within the tissue by a sharp and narrow region of high dose (the Bragg peak) at the end of the beam range that can be adjusted in that dimension to conform to the width of the target; and (III) very sharp lateral edges that can readily be made to conform to the projected cross-sectional contour of the target so that little or no dose is absorbed by the adjacent normal tissues.
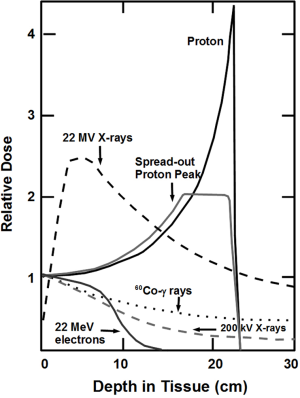
Synchrotron and/or cyclotron charged-particle accelerators are able to produce monoenergetic beams with ranges in tissue of 30 cm or more that can be modified precisely to specification by adjusting beam-output energy or by interposing energy-absorbing filters of appropriate design and thickness in the beam path, providing considerable flexibility in choice of beam directions for stereotactic treatment planning (19). Assuming exact knowledge of charged particle energy at the entry point and the physical properties of the intervening tissues, each charged-particle beam can be aimed stereotactically in 3D to place an individually shaped, high-dose region precisely within the brain. Beam ports from several coplanar or non-coplanar entry angles are selected to intersect within the target volume, resulting in a much lower dose to immediately adjacent and intervening normal brain tissues. Accurate prediction of charged particle range is therefore an important prerequisite for charged particle therapy, which makes the more uniform, motionless brain a prime application for charged particle radiosurgery.
Radiobiological properties of charged particles
An important topic of ongoing radiobiological interest has been the quality of ionization density along the particle trajectory, described by linear energy transfer (LET) as a function of particle velocity. The relative biological effectiveness (RBE) value at any given location in the path of the particle depends on the specific particle and its velocity at that location. Sparsely ionizing (low LET) radiation (with RBE values close to 1.0) is a property of all photon beams, as well as electrons with energies in the MeV range, and heavier charged particles such as pions, protons, and helium nuclei of high energy (>10 MeV per nucleon). Heavier ions (e.g., carbon) have higher ionization densities and RBE even at higher energies, which offer them a radiobiological advantage (RBE >2 in the Bragg peak region).
As protons (RBE=1.1) and helium ions (RBE=1.3) in the energy range used for radiosurgery have radiobiological properties very similar to photons, the primary advantage of using such particles is the ability to conform the delivered RT dose in 3D much more tightly to the designated target volume than is possible with photons. Therefore, expected normal tissue complications with these charged particles will be lower for a biologically equivalent dose to the target volume. Alternatively, a more aggressive, higher dose can be given to the target, while the dose to normal tissue can be kept at the same acceptable levels.
Some 15% to 20% of tumor histologies, however, are relatively radioresistant to low-LET irradiation, even at high doses. In these cases, the greater RBE of high-LET RT has shown great promise in the clinic and in the laboratory setting. Historically, neutrons were the first high-LET radiation used for many tumor types. Unfortunately, difficulties in conforming the neutron dose to the delineated target (i.e., adequately sparing critical adjacent normal tissues) severely limited the kinds of tumors for which this treatment could be applied. Beams of heavier charged particles (ranging from carbon to argon nuclei), however, exhibit high-LET radiation within their Bragg peaks, thereby integrating high RBE (about 3.0 for carbon) with excellent 3D dose conformity (20-22).
The radiobiological property underlying this RBE effect is that the higher-LET radiation is much more likely to cause clustered DNA damage, including double-strand breaks associated with additional breaks or base lesions in the targeted cells (23-25); by comparison, low-LET protons and X-ray beams typically cause more single-strand DNA breaks or double strand breaks that are not associated with additional DNA damage. The significance of complex double-strand DNA damage is that affected tumor cells are much less able to repair their DNA and survive high-LET treatment. This less-repairable DNA damage also appears to explain some other descriptions of enhanced cell death seen with high-LET RT that have been historically ascribed to the circumstances of reduced oxygen enhancement ratio or to less variation in sensitivity through the cell cycle (26-30). While normal cells may also suffer some double-strand damage with this technique, the preponderance of single-stranded and non-complex double-stranded DNA damage is much more repairable in the lower LET region of the plateau-ionization region where the normal tissues lie outside of the tumor target (31,32).
Another matter receiving increasing attention is the best method for integrating RBE information with the physical dose distribution. Historically, for simplicity, a simple RBE factor (1.1 for protons and 1.3 for helium nuclei) at a single reference point in the Bragg peak has been recorded. However, since heavier ions exhibit a wider range of RBE at different positions throughout the RT field, and protons and helium ions have an enhanced RBE at low energies (the last few millimeters before they stop), more detailed analysis is desirable. On a voxel-by-voxel basis, RBE values depend upon the variables of the specific ion used, dose per fraction, beam energy of the primary beam and its fragments, position within the Bragg-peak depth-dose curve, and the particular cell types or endpoints under consideration. In order to cope with this problem, the Local Effect Model (LEM), developed at GSI in Darmstadt, Germany, is a biophysical theory that attempts to incorporate these complex variables on a voxel-by-voxel basis, by integrating a non-linear response function over an inhomogeneous microscopic dose distribution (28,33,34). The required biological parameters for LEM include the experimental data on the response to sparsely ionizing radiation of the specific tumor and the adjacent normal tissues, and the size of the cell nucleus as the critical target. The required physical parameters include the atomic numbers of the primary beam and its nuclear fragments, and the velocity of the particles at different voxels in the field. These LEM calculations have been incorporated into the so-called “TRIP” treatment planning system for ion therapy, and have been used for 10 years with patients at GSI to calculate RBE values for all treated voxels, typically yielding local RBE values ranging from 2 to 4 in the target volume (35). While LEM has been applied extensively to carbon therapy at GSI with great success, it is now also being used to predict the incidence of late complications. LEM can also be adapted for use with low-LET particles, i.e., protons and helium ions, in order to predict the higher RBE values in the distal spread-out Bragg peak. The LEM-calculated treatment plan calculated to deliver a uniform biologically effective dose across a target volume typically requires a very heterogeneous physical dose delivery, unique for each beam-entry angle and fractional dose, mandating active beam-scanning dose delivery to accommodate the prescribed physical dose heterogeneity. Thus, very intensive computer calculation is required to optimize the LEM bio-effective treatment plan. A model similar to LEM has been developed in Japan by Kase et al. (36), based on the Microdosimetric Kinetic Model (MKM) of Hawkins that was elaborated from the theory of dual radiation action of Kellerer and Rossi (37). Both LEM and MKM conceptually follow some of the original ideas of Katz et al. (38). Two recent papers report the specification of carbon-ion doses at NIRS (39) and the treatment-planning strategy at Heidelberg (40).
Technique for charged-particle radiosurgery
Reliable stereotactic immobilization is required for both diagnostic imaging and millimeter-accurate dose delivery. It is imperative to reproduce both the translational coordinates (x, y, z), as well as the rotational degrees of pitch, yaw and roll, if complex target volumes are to be irradiated with adequate dose shaping. In the early era of charged-particle radiosurgery at LBNL, this was accomplished non-invasively using a thermoplastic mask to immobilize the patient within a relocatable stereotactic frame that was attached to the various imaging couches and then to the treatment-positioning table. The very first radiosurgical treatments in 1954 were performed at LBNL using protons, but in 1957 the Berkeley machine was modified to accelerate helium nuclei, and subsequent radiosurgery patients were treated with helium ions. As the mid-1950s were about 20 years before CT was invented, considerable uncertainty prevailed at that time in predicting particle beam range within the patients. Therefore, the radiosurgery method employed in those early years required that rigidly immobilized patients were treated with isocentrically intersecting arcs of high-energy proton and helium-ion beams that were used in a plateau shoot-through technique placing the Bragg peak beyond the patient’s distal surface, depositing that peak dose into the treatment table or into the walls of the treatment room—an early precursor to stereotactic photon radiosurgery (2).
At that time, accurate intracranial target delineation was limited to the pituitary gland, whose position within the midline structure of the sella turcica could be visualized readily by orthogonal plain radiographs. As increasingly sophisticated radiological imaging became available, image-fusion technology was developed to register cerebral angiograms and/or MRI with CT scans for improved target delineation of other intracranial lesions and for more-precise computerized treatment planning. Patient positioning verification for treatment was made by correlating digitally reconstructed radiographs with radio-opaque fiducial landmarks, such as adjacent bone structures. This immobilization technique was later supplemented at the Loma Linda University Medical Center (LLUMC) Proton Treatment Center by using vacuum-assisted dental fixation to assist the reliability of repositioning and by implanting titanium markers into the skull’s outer table for improved fiducial correlation with orthogonal radiographs for image guidance. Surgically attached stereotactic, cranial-halo reference frames have also been utilized, including frames that can be removed and reconnected days later to sockets that have been inserted into the outer table of the patient’s skull. At Harvard University, the Stereotactic Alignment System for Radiosurgery (STAR) was designed as an isocentric patient-positioning system with 6 degrees of freedom to enable a full spherical range of beam entry angles from a horizontal beam line (41). The STAR system has also proved to be an invaluable positioning device for fractionated proton treatment of cranial and skull base tumors. Based on the prior experience with advanced patient positioners for charged particle radiosurgery, all proton radiosurgery treatments at LLUMC are now performed with a robotic patient positioner that has 6 degrees of freedom.
Given the complexity of establishing accurate target delineation using image-fusion technology, and the multi-disciplinary evaluation required to optimize therapy recommendations, a strong argument can be made for using a relocatable stereotactic immobilization system. This relocatable frame approach also allows multi-fraction stereotactic treatment for larger and/or more eloquently located target volumes, as well as enabling multi-institutional collaboration. Additionally, removing the time-urgency of progressing to treatment permits a more thorough assessment of treatment planning options and quality-assurance testing.
Beginning in the mid-1970s with the advent of CT scanning, acquisition of voxel-specific, electron-density data (i.e., Hounsfield units) enabled more accurate depth-dose calculation of charged-particle stopping powers in heterogeneous tissue within the patients and heralded the dawn of Bragg peak radiosurgery with its much tighter dose conformity to intracranial target volumes. The narrow Bragg peak profile had to be adapted to the larger treatment volumes, initially using the so-called “spread out Bragg peak” (SOBP) technique developed at Berkeley and Harvard (42). SOBP’s were produced in analogous fashion to conventional photon therapy, using scattering foil systems, range-shifting absorbers, collimators, apertures and compensators to produce radiation fields that were more closely shaped to the target volume - but still not nearly as precisely as the 3D-conformity of modern beam-scanning techniques. Intracranial sites treated with radiosurgical technique expanded to include various benign tumors, as well as some primary and metastatic malignant tumors (43).
Although not traditionally categorized as representing stereotactic radiosurgery, charged-particle treatment of ocular diseases, including subfoveal macular degeneration and uveal melanoma, meets the definition of the term by requiring single- or limited-fraction external-beam irradiation of a target volume with millimeter accuracy. For these ocular lesions, treatment is given with a single fixed horizontal beam with the patient’s head immobilized while in an upright-seated position. Using a low-energy (typically, 70 MeV) proton beam, a single dose of 14-24 Gy (RBE) is applied to the retinal vascular abnormality of wet macular degeneration, and five fractions of a similar daily dose are applied to ocular melanoma (44). In each case, surgically placed metal clips at the posterior aspect of the globe serve as fiducial references for beams-eye-view X-ray alignment, with the eyelids held retracted by clips. With the patient staring at a precisely positioned light source, the margin of the iris is delineated with ink drawn onto a monitor screen whose video camera is focused on the surface of the eye, with the treatment beam immediately paused if eye movement is observed.
Dose-volume histogram analysis
3D treatment planning calculations have been used to compare the dose distributions for different techniques of stereotactic radiosurgery of intracranial target volumes, examining dose-volume histograms and integral doses to the target and to normal brain (45). In this analysis, the radiation doses to normal brain structures adjacent to and remote from the target volume were demonstrated to be relatively low with stereotactic charged-particle Bragg peak radiosurgery when compared with photon radiosurgery techniques that use focused beams of X-rays or gamma rays; this difference becomes especially marked in the treatment of larger intracranial lesions (Figure 2). Very similar conclusions were reached by other investigators performing comparative dose-volume studies (46,47).
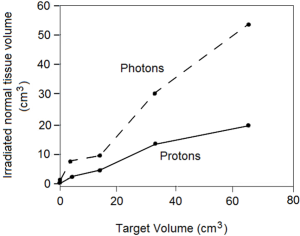
When irradiation of subfoveal neovascularization or ocular melanoma was evaluated by comparative analysis of the integral dose deposited in normal tissue, employing X-ray-based systems increased the integral dose to adjacent tissues (e.g., brain, pituitary gland) by a factor of 20 as compared to proton irradiation (48).
Clinical applications
A comprehensive review of the clinical experience and results in the field of charged-particle radiosurgery is beyond the scope of this brief review, and interested readers are referred to other manuscripts (1,2,43). Selected historically significant or representative studies have therefore been summarized and/or cited for further reference.
Pituitary gland
Charged-particle radiosurgery of the pituitary gland, historically its first clinical application, has proven to be a highly effective method for treatment of a variety of endocrine and metabolic hormone-dependent conditions, alone or in combination with surgical hypophysectomy and/or medical therapy in more than 3,500 patients worldwide. This includes patients with metastatic breast carcinoma and diabetic retinopathy, as well as hormone-secreting tumors such as acromegaly, Cushing’s disease, Nelson’s syndrome, and prolactin-secreting adenomas.
In the early years of the LBNL charged particle program, before anti-estrogen therapy became available, pituitary ablation for treatment of metastatic breast carcinoma was achieved with 180 to 270 Gy stereotactic proton or helium-ion plateau irradiation (2). Although this total dose was divided into six to eight fractions over two to three weeks, we consider this therapy to be radiosurgical in nature, as each individual treatment consisted of at least 30 Gy. Given the long-term pain relief from bone metastases that was achieved in many patients, this procedure was also employed with favorable results by proton centers in Moscow (49), Leningrad (50), and Boston (2). Pituitary ablation was also achieved with doses of 80 to 150 Gy for treatment of proliferative diabetic retinopathy, but with mixed clinical results (2,50,51).
For hormone-secreting tumors, before the development of trans-sphenoidal hypophysectomy, doses of 30 to 50 Gy were delivered in 3 or 4 fractions to the central core of the pituitary gland, while preserving a narrow rim of functional pituitary tissue. This treatment resulted in reliable control of tumor growth and suppression of hyper-secretion in a great majority of the patients. For example, mean serum growth hormone levels in a cohort of 234 patients with acromegaly treated with helium-ion radiosurgery decreased by nearly 70% in one year, continued to decrease thereafter, and remained normal throughout more than 10 years of follow-up (52).
Similar results were found with proton radiosurgery for acromegaly (10,53), and for treatment of Cushing’s disease and prolactinoma (2,11,54). Variable degrees of hypopituitarism occurred in as many as one-third of cases, but endocrine deficiencies were readily corrected with appropriate hormone supplemental therapy. The reader is referred to references (2) and (55) for comprehensive reviews of this subject.
Arteriovenous malformations
Charged-particle radiosurgery has also been applied to the treatment of intracranial AVMs in more than 3,000 patients worldwide since 1965 (2,13,14,16). While many AVMs are amenable to neurosurgical removal or endovascular embolization followed by surgery, surgical removal may involve high risks for the malformations located in deep or eloquent regions of the brain and for large lesions with multiple arterial supply or deep venous drainage. Moreover, these techniques, when possible, are not always completely successful because of the position or complexity of the malformation. The goal of radiosurgical treatment is to induce localized endothelial cell proliferation, vascular wall thickening, and thrombotic obliteration of the malformation while sparing normal adjacent brain structures. Given the complexity and variability of AVMs in terms of size and shape and the fact that many patients are children or young adults, the physical characteristics of charged-particle beams are uniquely advantageous for the radiosurgical treatment of these lesions. Bragg peak radiosurgery has the conformality required to treat eccentric and irregular AVMs of very large size (Figure 3), as well as to deliver sharp focal beams accurately to small lesions (e.g., in the brain stem or central nuclei) while protecting the adjacent critical nervous tissues and the rest of the normal brain (Figure 2) (1,2,16).
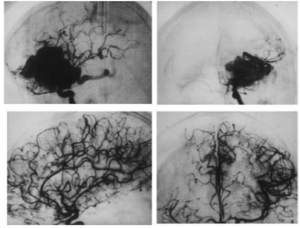
The most detailed report of charged-particle radiosurgery for AVM was that from the LBNL - Stanford University collaborative program (14). Here, 86 consecutive AVM patients (47 females, 39 males) ranging in age at the time of treatment from 9 to 69 years (mean, 33 years) were treated with helium-ion radiosurgery. Almost half of these patients (44%) had AVMs located in the brain stem, corpus callosum, thalamus, or basal ganglia, and most of the remainder had large malformations in eloquent areas of the cerebrum — the sensory, motor, language, or visual areas of the cortex. One-quarter of the AVMs were larger than 25 cm3.
In an evaluation carried out 24-72 months after radiosurgery, clinical outcome was graded as excellent in 58% and good in 36% of patients. In 63% of patients presenting with seizures and in 68% of patients presenting with headaches, there was an improvement in these symptoms. Two years after radiosurgery, angiographically demonstrated complete obliteration of the AVM occurred in 70%, partial closure (10% to 99% obliteration) occurred in 23%, and minimal or no change occurred in 7% of patients. Three years after treatment, 92% of patients had complete AVM obliteration, 4% had partial obliteration, and 4% experienced minimal or no change. The rate and extent of obliteration appeared to be a threshold phenomenon directly related to the AVM volume and the radiation dose. Smaller AVMs (<4 cm3) had higher rates of obliteration than larger ones and became thrombotic more rapidly and more completely than intermediate-sized (4 to 25 cm3) and larger (>25 cm3) lesions. However, full obliteration was observed 3 years following radiosurgery in 70% of AVMs >25 cm3.
Comparable results have been achieved with proton radiosurgery of AVMs after the LBNL-Stanford University helium radiosurgery program was transferred to LLUMC in 1993 and was continued there with protons. The experience with multimodality treatment (embolization and/or surgery performed at Stanford University and radiosurgery at LBNL or LLUMC) of very large (“giant”) AVMs was reported in 2003 (56). In 47 of 53 patients, radiosurgery was part of the multimodality treatment plan, and 40 of these patients were treated with helium ion or proton radiosurgery, while seven patients received photon (linear accelerator) radiosurgery. Of the 53 patients, 19 (36%) were cured, 4 (8%) achieved nearly complete (90%) obliteration, 29 (55%) had less than 90% obliteration; one patient was lost to follow-up. The long-term, treatment-related morbidity rate in this series was 15%. These results are remarkable, given that these large AVMs had been previously thought to be incurable at an acceptable rate of long-term morbidity. A multimodality approach with embolization, radiosurgery, and surgery as part of a staged procedure is probably necessary to achieve better results than those that can be accomplished with radiosurgery alone in these very large AVMs.
We recently analyzed the outcome of 40 patients with AVMs of small and intermediate size (<15 cm3) treated with proton radiosurgery alone at the LLUMC Proton Treatment Center. Patients were treated with a single or two fractions of proton radiosurgery to a dose of 25 Gy (RBE) to isocenter, corresponding to a marginal dose of 20 Gy (80%). The not-yet-published results of this analysis can be summarized as follows: out of the 29 patients with complete long-term imaging follow-up, the average AVM volume was 6.4 cm3 (range, 1-14.7 cm3). Complete angiographic obliteration rate was achieved in 69% (20/29). This includes 4 patients who failed their initial course of treatment and were successfully salvaged with a second course of proton radiosurgery, in which the dose was lowered to 20 Gy (RBE). The obliteration rates for AVMs <4 cm3 and 4-15 cm3 were 100% (9/9) and 55% (11/20), respectively. Two patients (7%) with AVMs larger than 10 cm3, including one after a second course of radiosurgery, developed a new onset of persistent weakness, which was attributed to the radiosurgery. All other patients had an excellent neurological outcome.
Additional single-institution reports of small series of AVMs treated with proton radiosurgery have appeared in the literature over the last 10 years, and the reader is referred to references (57-59) for additional information on this subject.
Brain metastases
At LLUMC and at other proton facilities, protocols have been developed and implemented for stereotactic radiosurgery of brain metastases, a condition with an annual incidence in the U.S. similar to that of primary prostate cancer. Most patients treated with protons at LLUMC for this condition are individuals who were first treated for their primary disease in our department and then developed secondary metastases subsequently or during their primary treatment.
Indications for proton radiosurgery of brain metastases in the LLUMC experience are adjuvant treatment of remaining metastases immediately following whole-brain radiation therapy (WBRT) and/or surgery (30%), salvage treatment for new or residual brain metastases more than one month after the primary treatment for metastatic disease (50%), and radiosurgery as the primary treatment modality, mostly for single or solitary metastases to the brain (20%). The Kaplan-Meyer survival curves of the first 46 consecutive patients with brain metastases treated at LLUMC are shown in Figure 4. On multivariate analysis, single versus multiple metastases at the time of treatment was the only prognostic factor associated with a better survival outcome (median survival: 15 versus 9 months, P<0.01).
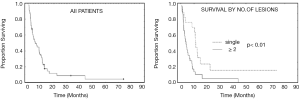
Of interest, there were two long-term survivors in the LLUMC series of 46 patients. Long-term survival has also been reported in a single-institution report from the Cleveland Clinic including 1,288 patients diagnosed with brain metastases and treated with WBRT, surgery, and/or stereotactic radiosurgery (60). In that series thirty-two patients (2.5%) survived more than 5 years.
In the LLUMC experience, the use of proton radiosurgery for brain metastases can be of advantage if the patent returns for retreatment of a single metastasis or oligo-metastatic disease a few months after the first radiosurgery treatment. This scenario is more common now that primary radiosurgery is being practiced more frequently due to the fear of mental decline after WBRT. Figure 5 shows the MRI of a patient treated consecutively for two asynchronous metastases with an interval of 6 months between the two treatments. Due to the proximity of the two metastases, the lack of dose beyond the Bragg peak was clearly an advantage for the repeated radiosurgery.
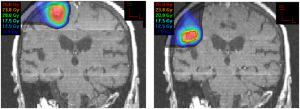
Benign tumors
At LLUMC, various benign tumors, including pituitary adenomas, acoustic neuromas and meningiomas have been successfully treated by protons with conventional fractionation schedules [1.8 Gy (RBE) per fraction] (61-63). For all tumor histologies, long-term radiological local control rates were higher than 90%. Good outcomes with local control rates consistently above 90%, and thus comparable to the results with conventional fractionation, have also been achieved following charged-particle radiosurgery with 1-3 fractions for acoustic neuromas (64,65) and a single dose for meningiomas (66).
Related to hearing preservation, the question whether acoustic neuromas are better treated with stereotactic radiation therapy rather than radiosurgery remains open as various groups have been struggling with defining the best dose that leads to an acceptable hearing preservation with either technique. For example, the investigators at the Massachusetts General Hospital (MGH) reduced their maximum single-fraction proton radiosurgery dose to the target volume from 17.1 Gy (RBE) down to 13.3 Gy (RBE) in 1999 (64). In their 2003 review, only one of five patients treated at either dose retained functional hearing with longer audiometric follow-up (64). The authors concluded that patients with acoustic neuromas are better treated with fractionated stereotactic RT than single-dose radiosurgery. At LLUMC, only 31% of patients with initially useful hearing retained it during a mean follow-up period of 34 months after fractionated RT to 54 Gy (RBE) (62). A newer but not-yet-published analysis from the same institution shows a clear trend of improved hearing preservation after the dose had been reduced to 50.4 Gy (RBE) with 1.8 Gy per fraction, but longer follow-up will be needed to confirm that the high rates of local control are maintained.
Ocular irradiation - Age-related macular degeneration
Proton-beam irradiation for the “wet” form of age-related macular degeneration (AMD), which is associated with subfoveal neovascularization, showed favorable initial results in a small Phase I/II trial with a single dose of 8 Gy (RBE) performed at LLUMC (67). The rationale for this treatment was based on the observed sensitivity to irradiation of proliferating endothelial cells in culture (68), and the relative resistance to irradiation of the retina and choroid. Proton irradiation is ideally suited for this task, since a satisfactory dose to the neovascular membrane can be delivered in a few minutes, using a single anterior oblique proton field to deliver a therapeutic dose. The proton beam stops without entering the brain or reaching the opposite eye. Subsequent results of a non-randomized study comparing the efficacy of a single dose of 8 Gy (RBE) to a single dose of 14 Gy (RBE) in 21 and 27 patients, respectively, showed that a dose of 14 Gy (RBE) was more effective in halting visual loss: actuarial lesion control at 21 months was 36% for 8-Gy (RBE) patients and 89% for 14-Gy (RBE) patients (69). However, 11 eyes in the 14 Gy group experienced some radiation retinopathy, with the onset between 3 and 30 months, although only one patient developed a severe visual loss at 15 months after proton treatment (70).
Ciulla and colleagues (71) examined the effect of proton irradiation on neovascular membranes associated with AMD in a randomized, prospective, sham-controlled, double-blind study. Thirty-seven patients were randomly assigned to 16 Gy (RBE) proton irradiation delivered in two equal fractions or to sham control treatment. However, recruitment was stopped after 37 patients had been enrolled, citing ethical reasons regarding randomization to sham treatment when FDA approval of the photosensitizer Visudyne in combination with photodynamic laser therapy was anticipated. When the results in the control and proton irradiation were analyzed, proton irradiation was associated with a trend toward stabilization of visual acuity, without reaching statistical significance.
Another randomized controlled trial (n=166) conducted at the MGH (72) evaluated the safety and visual outcomes after proton therapy to either 16 Gy (RBE) or 24 Gy (RBE) for subfoveal neovascular patients with AMD in two equal fractions. The investigators found no significant differences in rates of visual acuity or complications between the two study arms, but suggested that proton beam therapy may be useful as an adjuvant therapy or as an alternative for patients who decline or are not appropriate for approved therapies.
Ocular irradiation - Uveal melanomas
Episcleral radionuclide plaque brachytherapy and charged-particle RT are established eye- and vision-sparing alternatives to enucleation in patients with uveal melanomas (73). At LLUMC and other centers with proton eye-beam lines, 14 Gy (RBE) (the dose used for subfoveal neovascularization in a single fraction, as described above) is given five times for a total dose of 70 Gy (RBE) for treatment of uveal melanomas. About 12,000 patients worldwide have now been treated using this scheme of hypo-fractionated proton therapy for uveal melanomas, and local tumor control rates in excess of 95% (in smaller tumors) have been achieved consistently for this lesion that is generally considered to be radiation resistant to standard fractionation.
Charged-particle RT has been performed with proton beams (74) and helium ion beams (20) since the mid-1970s. In 1988, Munzenrider and colleagues from the MGH (75) reported on the outcomes in 1006 uveal melanoma patients treated with proton beams at the Harvard Cyclotron. Eye retention rates at 60 months were 89.1±3.0% for the entire group, and 97±3.7%, 92.7±3.1%, and 78.3±7.0% in patients with small, intermediate, and large tumors, respectively. Significantly greater enucleation rates were subsequently observed in patients with large tumors than in those with intermediate tumors (P≤0.0001), in patients with tumor height >8 mm relative to those with tumors ≤8 mm (P≤0.0001), with tumor diameter >16 mm compared to ≤16 mm, (P≤0.0001), and with tumor involvement of the ciliary body compared to involvement of the choroid only (P≤0.0001).
A separate analysis of 562 patients with pretreatment visual acuity of 20/200 or better, treated at the Harvard Cyclotron over a 10-year period, investigated prognostic factors for visual loss after proton radiosurgery to 70 Gy (RBE) in 5 fractions (76). Of 562 eyes, 363 (64.6%) contained tumors within 2 disc diameters (DD) of the disc or fovea. Two-year actuarial rates of loss of useful vision (worse than 20/200) among patients with tumors near the disc or fovea were 47%, compared with 28% for patients with tumors located farther from both structures.
Seeking to determine whether reducing the proton dose from 70 Gy (RBE) to 50 Gy (RBE) would decrease radiation-induced complications without compromising local control, the MGH/Harvard team subsequently conducted a randomized, double-blind study in 188 patients with small or medium-sized choroidal melanomas (<15 mm in diameter and <5 mm in height) within 4 DD of the optic disc or macula (77). Although the proportion (55%) of patients retaining visual acuity (VA) of at least 20/200, and the local and metastatic failure rates, were similar in the two dose groups at 5 years, patients treated to a lower dose had significantly less visual field loss.
In Europe, the largest experience in treating uveal melanomas with protons has been accumulated at the Paul Scherrer Institute in Switzerland starting in 1984. In 1999, the treatment outcomes of 2,435 uveal melanomas in 2,432 patients treated with proton beam RT between March 1984 and December 1998 were analyzed (78). The median follow-up time was 40 months. Initially, treatment had started with the application of 70 Gy (RBE) in 4 fractions, which was subsequently lowered to 65 Gy, then to 63 Gy, and finally to 60 Gy. At the time of analysis, most patients had been treated with 60 Gy (RBE) in 4 fractions. The largest tumor diameter ranged from 4 to 26 mm, and tumor thickness ranged from 0.9 to 15.6 mm. There was a trend to improved local control at 5 years over time from 90.6±1.7% for patients treated before 1988 to 98.9±0.6% for patients treated after 1993, which was attributed to changes in the treatment procedure. Of the 2,435 patients, 73 (3%) required a second treatment (proton beam RT, enucleation or brachytherapy) for tumor regrowth. Cause-specific survival at 10 years was 72.6±1.9% for patients with controlled tumors compared with 47.5±6.5% for those with recurrent tumors. The main prognostic factors for reduced local tumor control were large ciliary body tumors and male gender as well as technical factors, including eyelids within the treatment field and inadequate positioning of tantalum clips for tumor localization before treatment. In a later analysis (79), the overall eye retention rates at 5, 10, and 15 years after treatment were reported as 88.9%, 86.2%, and 83.7%, respectively. Enucleation was related to larger tumor size (mainly tumor height), proximity of posterior tumor margin to optic disc, male gender, high intraocular pressure, and large degree of retinal detachment at treatment time.
Similar results were also reported after helium-ion RT for uveal melanomas. Char et al. prospectively investigated 218 patients treated at LBNL between 1978 and 1984 (80). Ten years following treatment, 46 eyes (22.4%) had been enucleated, and most enucleations (37 of 46) were due to anterior-segment ocular complications. The overall, 10-year local control rate was 95.4%, but 51 patients (23.4%) died from metastatic disease. For patients with tumors less than 6 mm in height and more than 3 mm distant from the optic nerve or fovea, 13 of 18 (72%) retained VA greater than 20/40. By contrast, only 11% of the patients that did not meet these criteria retained a good level of VA. The same group of investigators also reported on a prospective trial in which patients with uveal melanoma were randomized to receive helium-ion irradiation (n=86) or iodine-125 (n=98) brachytherapy (81). Tumor diameter was <15 mm and tumor thickness <10 mm in all patients. Local recurrence rate after 125I brachytherapy was significantly higher than after helium-ion irradiation (13% vs. 0%). Enucleation was also required more frequently after brachytherapy (relative risk =1.99; 95% confidence interval, 0.78-5.78), but more anterior-segment complications occurred following helium-ion RT, and there was no difference in overall survival.
At LLUMC, a retrospective review of 78 patients with 60 medium-sized (base <16 mm, apex 3-10 mm) and 18 large-sized (base ≥16 mm, apex ≥10 mm) choroidal melanomas was performed in the early 2000s (82). With a median follow-up of 34 months, the 5-year local control rate was 90.5±3.7%, and metastases-free and disease-specific survival rates were 76.2±6.7% and 75.6±7.6%, respectively. Eye retention was achieved in 75.3% and useful (better than 20/200) VA persisted in 49.1% of surviving patients. Prognostic factors for loss of the affected eye due to complications were close proximity to the optic disc (P=0.003) and large tumors involving the ciliary body (P=0.041). Prognostic factors for VA outcome were initial VA (P=0.001), doses to optic disc (P=0.001) and fovea (P=0.022) higher than 35 Gy (RBE), tumor proximity to the optic disc (P=0.034), and retinal detachment (P<0.001). Diameter of the tumor base was significantly related to metastases-free survival (P=0.02) and overall survival (P=0.033), but that did not impact local control, eye retention, or VA.
Other large series of uveal melanomas treated with 5 fractions of proton beam RT were reported by the centers in Nice, France (83), and Clatterbridge, UK (84), and the interested reader is referred to their reports.
Summary
Stereotactic charged-particle radiosurgery has now been successfully employed for almost 60 years in the clinical treatment of more than 15,000 intracranial patients and 12,000 ocular patients. Treated disorders include pituitary tumors, vascular malformations, primary and metastatic malignant brain tumors, various other benign brain tumors, uveal melanomas, and subfoveal neovascularization. The unique physical properties of charged particles make this method particularly advantageous for the conformal treatment of large and/or irregularly shaped lesions or for the treatment of lesions located in front of or adjacent to sensitive brain structures.
The role of heavier charged particles (e.g., carbon nuclei) as high-LET, radiosurgical boost therapy for the treatment of radioresistant brain tumors is the subject of ongoing investigation. Technological improvements in charged particle therapy described elsewhere in this edition of Translational Cancer Research are likely to lead to further applications and improved outcomes in charged particle radiosurgery.
Acknowledgments
Funding: None.
Footnote
Provenance and Peer Review: This article was commissioned by the Guest Editors (Huan Giap and Eric Y Chuang) for the series “Particle Beam Therapy I” published in Translational Cancer Research. The article has undergone external peer review.
Conflicts of Interest: Both authors have completed the ICMJE uniform disclosure form (available at http://dx.doi.org/10.3978/j.issn.2218-676X.2012.10.04). The series “Particle Beam Therapy I” was commissioned by the editorial office without any funding or sponsorship. RPL and RWMS serves as the unpaid editorial board members of Translational Cancer Research. The authors have no potential conflicts of interest related to the information contained herein.
Ethical Statement: The authors are accountable for all aspects of the work in ensuring that questions related to the accuracy or integrity of any part of the work are appropriately investigated and resolved.
Open Access Statement: This is an Open Access article distributed in accordance with the Creative Commons Attribution-NonCommercial-NoDerivs 4.0 International License (CC BY-NC-ND 4.0), which permits the non-commercial replication and distribution of the article with the strict proviso that no changes or edits are made and the original work is properly cited (including links to both the formal publication through the relevant DOI and the license). See: https://creativecommons.org/licenses/by-nc-nd/4.0/.
References
- Fabrikant JI, Levy RP, Steinberg GK, et al. Charged-particle radiosurgery for intracranial vascular malformations. Neurosurg Clin N Am 1992;3:99-139. [PubMed]
- Levy RP, Fabrikant JI, Frankel KA, et al. Charged-particle radiosurgery of the brain. Neurosurg Clin N Am 1990;1:955-90. [PubMed]
- Wilson RR. Radiological use of fast protons. Radiology 1946;47:487-91. [PubMed]
- Brobeck WM, Lawrence EO, MacKenzie KR, et al. Initial performance of the 184 inch cyclotron of the University of California. Phys Rev 1947;71:449-50.
- Tobias CA, Anger HO, Lawrence JH. Radiologic use of high energy deuterons and alpha particles. Am J Roentgenol Radium Ther Nucl Med 1952;67:1-27. [PubMed]
- Rodriguez A, Levy RP, Fabrikant JI. Experimental central nervous system injury after charged-particle irradiation. In: Gutin PH, Leibel SA, Sheline GE. eds. Radiation Injury to the Nervous System. New York: Raven Press, 1990:149-82.
- Lawrence JH. Proton irradiation of the pituitary. Cancer 1957;10:795-8. [PubMed]
- Lawrence JH, Tobias CA, Born JL, et al. Heavy-particle irradiation in neoplastic and neurologic disease. J Neurosurg 1962;19:717-22. [PubMed]
- Lawrence JH, Tobias CA, Linfoot JA, et al. Heavy particles, the Bragg curve and suppression of pituitary function in diabetic retinopathy. Diabetes 1963;12:490-501. [PubMed]
- Kjellberg RN, Shintani A, Franzt AG, et al. Proton beam therapy in acromegaly. N Engl J Med 1968;278:689-95. [PubMed]
- Linfoot JA, Lawrence JH, Born JL, et al. The alpha particle or proton beam in radiosurgery of the pituitary gland for Cushing’s disease. N Engl J Med 1963;269:597-601. [PubMed]
- Fabrikant JI, Lyman JT, Hosobuchi Y. Stereotactic heavy-ion Bragg peak radiosurgery: method for treatment of deep arteriovenous malformations. Br J Radiol 1984;57:479-90. [PubMed]
- Kjellberg RN, Hanamura T, Davis KR, et al. Bragg peak proton-beam therapy for arteriovenous malformations of the brain. N Engl J Med 1983;309:269-74. [PubMed]
- Steinberg GK, Fabrikant JI, Marks MP, et al. Stereotactic heavy-charged particle Bragg peak radiation for intracranial arteriovenous malformations. N Engl J Med 1990;323:96-101. [PubMed]
- Phillips MH, Singh RP, Castro JR, et al. Treatment planning for heavy charged particle radiotherapy. Int J Radiat Oncol Biol Phys 1991;20:881-9. [PubMed]
- Chen GT, Kessler M, Chuang FYS, et al. Image correlation of MRI and CT in treatment planning for radiosurgery of intracranial vascular malformations. Int J Radiat Oncol Biol Phys 1979;5:1809-19. [PubMed]
- Giap FN, Levy RP, Giap HB. Summary of ongoing clinical protocols for proton and heavier ion therapy. Am Inst Phys Conference Proceedings. Proceedings the 22nd International Conference on the Application of Accelerators in Research and Industry (CAARI) 2012. In press by American Institute of Physics Conference Proceedings Series 2012.
- Bragg WH, Kleeman R. On the ionization curve of radium. Philos Mag 1904;S6:726-38.
- Levy RP, Fabrikant JI, Frankel KA, et al. Stereotactic heavy-charged-particle Bragg peak radiosurgery for the treatment of intracranial arteriovenous malformations in childhood and adolescence. Neurosurgery 1989;24:841-52. [PubMed]
- Castro JR, Quivey JM, Lyman JT, et al. Current status of clinical particle radiotherapy at Lawrence Berkeley Laboratory. Cancer 1980;46:633-41. [PubMed]
- Blakely EA. Current issues in low and high LET medical radiobiology. In: Amaldi U, Larsson B. eds. Hadrontherapy in Oncology. Paris, Iune: Int Congr Series. Elsevier Science,1994:693-701.
- Kraft G. Tumor therapy with heavy charged particles. Progr Part Nucl Phys 2000;45:473-544.
- Roots R, Holley W, Chaterjee A, et al. The formation of strand breaks in DNA after high-LET irradiation: a comparison of data from in vitro and cellular systems. Int J Radiat Biol 1990;58:55-69. [PubMed]
- Hirayama R, Furusawa Y, Fukawa T, et al. Repair kinetics of DNA-DSB induced by X-rays or carbon ions under oxic and hypoxic conditions. J Radiat Res (Tokyo) 2005;46:325-32. [PubMed]
- Hada M, Georgakilas AG. Formation of clustered DNA damage after high-LET irradiation: a review J Radiat Res 2008;49:203-10. [PubMed]
- Blakely EA, Ngo FQH, Curtis SB, et al. Heavy-ion radiobiology: cellular studies. Adv Radiat Biol 1984;11:295-389.
- Tauchi H, Komatsu K. Cell cycle and LET dependence for radiation-induced mutation: a possible mechanism for reversed dose-rate effect. J Radiat Res (Tokyo) 1999;40:45-52. [PubMed]
- Scholz M, Kraft G. Calculation of heavy ion inactivation probabilities based on track structure, X-ray sensitivity and target size. Radiat Prot Dosim 1994;52:29-33.
- Blakely EA, Chang PY, Lommel L. Cell-cycle-dependent recovery from heavy-ion damage in G1-phase cells. Radiat Res Suppl 1985;8:S145-57. [PubMed]
- Lücke-Huhle C, Blakely EA, Chang PY, et al. Drastic G2 arrest in mammalian cells after irradiation with heavy-ion beams. Radiat Res 1979;79:97-112. [PubMed]
- Ando K, Koike S, Uzawa A, et al. Repair of skin damage during fractionated irradiation with gamma rays and low-LET carbon ions. J Radiat Res (Tokyo) 2006;47:167-74. [PubMed]
- Blakely EA, Kronenberg A. Heavy-ion radiobiology: new approaches to delineate mechanisms underlying enhanced biological effectiveness. Radiat Res 1998;150:S126-45. [PubMed]
- Elsässer T, Scholz M. Improvement of the local effect model (LEM) --- implications of clustered DNA damage. Radiat Prot Dosimetry 2006;122:475-7. [PubMed]
- Elsässer T, Krämer M, Scholz M. Accuracy of the Local Effect Model for the prediction of biologic effects of carbon ion beams in-vitro and in-vivo. Int J Radiat Oncol Biol Phys 2008;71:866-72. [PubMed]
- Krämer M, Jäkel O, Haberer T, et al. Treatment planning for heavy-ion radiotherapy: physical beam model and dose optimization. Phys Med Biol 2000;45:3299-317. [PubMed]
- Kase Y, Kanai T, Matsufuji N, et al. Biophysical calculation of cell survival probabilities using amorphous track structure models for heavy-ion irradiation. Phys Med Biol 2008;53:37-59. [PubMed]
- Kellerer AM, Rossi HH. A generalized formulation of dual radiation action. Radiat Res 2012;178:AV204-13. [PubMed]
- Katz R, Anderson B, Homayoonfar M, et al. Inactivation of cells by heavy ion bombardment. Radiat Res 1971;47:402-25. [PubMed]
- Matsufuji N, Kanai T, Kanematsu N, et al. Specification of carbon ion dose at the National Institute of Radiological Sciences (NIRS). J Radiat Res 2007;48:A81-6. [PubMed]
- Jäkel O, Schulz-Erner D, Debus J. Specifying carbon ion doses for radiotherapy: the Heidelberg approach. J Radiat Res 2007;48:A87-95. [PubMed]
- Chapman P. The STAR radiosurgery program at Harvard and MGH- the successful optimization of resources at two facilities. PTCOG48; Heidelberg, Germany, 2009.
- Chu WT, Ludewigt BA, Renner TR. Instrumentation for treatment of cancer using proton and light-ion beams. Rev Sci Instr 1993;64:2055-122.
- Harsh G, Loeffler JS, Thornton A, et al. Stereotactic proton radiosurgery. Neurosurg Clin N Am 1999;10:243-56. [PubMed]
- Gragoudas E, Li W, Goitein M, et al. Evidence-based estimates of outcome in patients treated for intraocular melanoma. Arch. Ophthalmol 2002;120:1665-71. [PubMed]
- Phillips MH, Frankel KA, Lyman JT, et al. Comparison of different radiation types and irradiation geometries in stereotactic radiosurgery. Int J Radiat Oncol Biol Phys 1990;18:211-20. [PubMed]
- Verhey LJ, Smith V, Serago CF. Comparison of radiosurgery treatment modalities based on physical dose distributions. Int J Radiat Oncol Biol Phys 1998;40:497-505. [PubMed]
- Baumert BG, Lomax AJ, Miltchev V, et al. A comparison of dose distributions of proton and photon beams in stereotactic conformal radiotherapy of brain lesions. Int J Radiat Oncol Biol Phys 2001;49:1439-49. [PubMed]
- Moyers MF, Galindo RA, Yonemoto LT, et al. Treatment of macular degeneration with proton beams. Med Phys 1999;26:777-82. [PubMed]
- Minakova EI, Vasil’eva NN, Svyatukhina OV. Irradiation of the hypophysis with single large dose of high energy protons for advanced breast carcinoma Med Radiol (Mosk) 1977;22:33-9. (in Russian). [PubMed]
- Konnov B, Melnikov L, Zargarova O, et al. Narrow proton beam therapy for intracranial lesions. In: Heikkinen E, Kiviniitty K. eds. International Workshop on Proton and Narrow Photon Beam Therapy. Oulu, Finland, University of Oulu Printing Center, 1989:48-55.
- Kjellberg RN, McMeel JW, McManus NL, et al. Pituitary suppression in diabetic retinopathy by proton beam in surgically “unfit” patients. In: Goldberg MF, Fine SL. eds. Symposium on the Treatment of Diabetic Retinopathy. Arlington: US Public Health Service Publication No. 1890. 1968:249-76.
- Lawrence JH, Linfoot JA. Treatment of acromegaly, Cushing disease and Nelson syndrome. West J Med 1980;133:197-202. [PubMed]
- Petit JH, Biller BMK, Coen JJ, et al. Proton stereotactic radiosurgery in management of persistent acromegaly. Endocr Pract 2007;13:726-34. [PubMed]
- Petit JH, Biller BM, Yock TI, et al. Proton stereotactic radiotherapy for persistent adrenocorticotropin-producing adenomas. J Clin Endocrinol Metab 2008;93:393-9. [PubMed]
- Levy RP, Fabrikant JI. Clinical applications of stereotactic radiosurgery. In: Phillips MH. eds. Physical Aspects of Stereotactic Radiosurgery. New York: Plenum, 1993:239-78.
- Chang SD, Marcellus ML, Marks MP, et al. Multimodality treatment of giant intracranial arteriovenous malformations. Neurosurgery 2003;53:1-11. [PubMed]
- Vernimmen FJ, Slabbert JP, Wilson JA, et al. Stereotactic proton beam therapy for intracranial arteriovenous malformations. Int J Radiat Oncol Biol Phys 2005;62:44-52. [PubMed]
- Ito Y, Okumura T, Suzuki K, et al. Long-term outcome of proton beam radiosurgery for arteriovenous malformations larger than 30 mm in diameter. Neurol Med Chir (Tokyo) 2011;51:624-9. [PubMed]
- Hattangadi JA, Chapman PH, Bussière MR, et al. Planned two-fraction proton beam stereotactic radiosurgery for high-risk inoperable cerebral arteriovenous malformations. Int J Radiat Oncol Biol Phys 2012;83:533-41. [PubMed]
- Chao ST, Barnett GH, Liu SW, et al. Five-year survivors of brain metastases: a single-institution report of 32 patients. Int J Radiat Oncol Biol Phys 2006;66:801-9. [PubMed]
- Ronson BB, Schulte RW, Han KP, et al. Fractionated proton beam irradiation of pituitary adenomas. Int J Radiat Oncol Biol Phys 2006;64:425-34. [PubMed]
- Bush DA, McAllister CJ, Loredo LN, et al. Fractionated proton beam radiotherapy for acoustic neuroma. Neurosurgery 2002;50:270-3. [PubMed]
- Slater JD, Loredo LN, Chung A, et al. Fractionated proton radiotherapy for benign cavernous sinus meningiomas. Int J Radiat Oncol Biol Phys 2012;83:e633-7. [PubMed]
- Weber DC, Chan AW, Bussiere MR, et al. Proton beam radiosurgery for vestibular schwannoma: tumor control and cranial nerve toxicity. Neurosurgery 2003;53:577-86. [PubMed]
- Vernimmen FJ, Mohamed Z, Slabbert JP, et al. Long-term results of stereotactic proton beam radiotherapy for acoustic neuromas. Radiother Oncol 2009;90:208-12. [PubMed]
- Halasz LM, Bussière MR, Dennis ER, et al. Proton stereotactic radiosurgery for the treatment of benign meningiomas. Int J Radiat Oncol Biol Phys 2011;81:1428-35. [PubMed]
- Yonemoto LT, Slater JD, Friedrichsen EJ, et al. Phase I/II study of proton beam irradiation for the treatment of subfoveal choroidal neovascularization in age-related macular degeneration: treatment techniques and preliminary results. Int J Radiat Oncol Biol Phys 1996;36:867-71. [PubMed]
- Mao XW, Archambeau JO, Kubínová L, et al. Quantification of rat retinal growth and vascular population changes after single and split doses of proton irradiation: translational study using stereology methods. Radiat Res 2003;160:5-13. [PubMed]
- Yonemoto LT, Slater JD, Blacharski P, et al. Dose response in the treatment of subfoveal choroidal neovascularization in age-related macular degeneration: results of a phase I/II dose-escalation study using proton radiotherapy. J Radiosurgery 2000;3:47-54.
- Flaxel CJ, Friedrichsen EJ, Smith JO, et al. Proton beam irradiation of subfoveal choroidal neovascularisation in age-related macular degeneration. Eye (Lond) 2000;14:155-64. [PubMed]
- Ciulla TA, Danis RP, Klein SB, et al. Proton therapy for exudative age-related macular degeneration: a randomized, sham-controlled clinical trial. Am J Ophthalmol 2002;134:905-6. [PubMed]
- Zambarakji HJ, Lane AM, Ezra E, et al. Proton beam irradiation for neovascular age-related macular degeneration. Ophthalmology 2006;113:2012-9. [PubMed]
- Shields CL, Shields JA, Gündüz K, et al. Radiation therapy for uveal malignant melanoma. Ophthalmic Surg Lasers 1998;29:397-409. [PubMed]
- Gragoudas ES, Goitein M, Koehler AM, et al. Proton irradiation of small choroidal malignant melanomas. Am J Ophthalmol 1977;83:665-73. [PubMed]
- Munzenrider JE, Gragoudas ES, Seddon JM, et al. Conservative treatment of uveal melanoma: probability of eye retention after proton treatment. Int J Radiat Oncol Biol Phys 1988;15:553-8. [PubMed]
- Seddon JM, Gragoudas ES, Egan KM, et al. Uveal melanomas near the optic disc or fovea. Visual results after proton beam irradiation. Ophthalmology 1987;94:354-61. [PubMed]
- Gragoudas ES, Lane AM, Regan S, et al. A randomized controlled trial of varying radiation doses in the treatment of choroidal melanoma. Arch Ophthalmol 2000;118:773-8. [PubMed]
- Egger E, Schalenbourg A, Zografos L, et al. Maximizing local tumor control and survival after proton beam radiotherapy of uveal melanoma. Int J Radiat Oncol Biol Phys 2001;51:138-47. [PubMed]
- Egger E, Zografos L, Schalenbourg A, et al. Eye retention after proton beam radiotherapy for uveal melanoma. Int J Radiat Oncol Biol Phys 2003;55:867-80. [PubMed]
- Char DH, Kroll SM, Castro J. Long-term follow-up after uveal melanoma charged particle therapy. Trans Am Ophthalmol Soc 1997;95:171-87. [PubMed]
- Char DH, Quivey JM, Castro JR, et al. Helium ions versus iodine 125 brachytherapy in the management of uveal melanoma. A prospective, randomized, dynamically balanced trial. Ophthalmology 1993;100:1547-54. [PubMed]
- Fuss M, Loredo LN, Blacharski PA, et al. Proton radiation therapy for medium and large choroidal melanoma: preservation of the eye and its functionality. Int J Radiat Oncol Biol Phys 2001;49:1053-9. [PubMed]
- Caujolle JP, Mammar H, Chamorey E, et al. Proton beam radiotherapy for uveal melanomas at Nice teaching hospital: 16 years’ experience. Int J Radiat Oncol Biol Phys 2010;78:98-103. [PubMed]
- Wilson MW, Hungerford JL. Comparison of episcleral plaque and proton beam radiation therapy for the treatment of choroidal melanoma. Ophthalmology 1999;106:1579-87. [PubMed]