Shuttling towards a predictive assay for radiotherapy
Acute and late effects such as moist desquamation, radiation enteropathy, and radiation-induced secondary malignancy, which occur in up to 20% of patients, constitute major drawbacks to radiation therapy. As a result, many studies have attempted to identify individuals who are likely to suffer these effects prior to therapy (1). Many of the studies have focused on DNA damage and repair because of their strong association with cell survival. However, to date no assays have been developed that reliably predict patient response.
A recent report from the COPERNIC project investigators (2), describes the development of an immunofluorescence assay where patient-derived fibroblasts are probed with antibodies to phosphorylated ataxia telangiectasia mutated (pATM) kinase and a key substrate, phosphorylated histone H2AX (γ-H2AX), to predict the radiation sensitivity of patients undergoing radiation therapy. The study made use of 100 untransformed fibroblast lines belonging to the COPERNIC collection that were derived from patients who had suffered moderate adverse effects following radiation therapy (based on the severity grades established by the Common Terminology Criteria for Adverse Events and the Radiation Therapy Oncology Group), in addition to fibroblasts from radioresistant and hyperradiosensitive individuals. The authors found that both γ-H2AX and pATM foci could be quantified and, together, predict human radiosensitivity. This is interesting because it implies that, despite γ-H2AX being a downstream target of the ATM kinase, the two antibodies provide at least partially independent information. Importantly, while their approach clearly identified hypersensitive cell lines with overt mutations in genes required for DNA repair, it was also able to resolve an intermediate response that correlated with moderate radiosensitivity. Specifically, they find that the antibody generated against phosphorylated ATM shows a strong cytoplasmic signal and a weaker nuclear signal that increases with the number of radiation-induced DNA double-strand breaks (DSBs). The rate of accumulation of the pATM signal in nuclear foci appears to be the determining factor and they suggest that this reflects patient-specific differences in the trafficking of pATM from the cytoplasm to the nucleus, which in turn determines DSB recognition and DNA repair in the nucleus. In cells derived from radiosensitive patients, pATM and γ-H2AX foci formation in the nuclei of irradiated cells is delayed relative to radioresistant patients.
To perform this assay, a punch biopsy is collected, skin fibroblasts are isolated, and then grown in culture prior to testing. After the fibroblasts have been isolated and expanded in culture, they are irradiated with 2 Gy and then analyzed at 10-minute or 24-hour time points. The irradiation is carried out with cells in the plateau phase of growth to mimic healthy tissues and to avoid any variation that reflects differences in cell cycle distribution. While the assay could be implemented in a clinical setting, the assay will be difficult and expensive to implement because of the need to isolate and grow patient fibroblasts. Consequently, it is worth exploring what we know about the mechanisms that the authors have implicated as the basis for the predictive power of their assay and ask whether or not there are additional potential biomarkers of this response that would be amenable to a simplified assay of patient tissue directly.
Ataxia telangiectasia mutated (ATM) in the cytoplasm?
ATM is a protein kinase and a member of the evolutionarily conserved phosphatidylinositol-3-kinase related kinase (PIKK) family (3). It is a large protein with a molecular weight of 350 kDa and consists of 3,056 amino acids (4). Patients with ATM deficiency are affected by the human autosomal recessive disorder ataxia telangiectasia (A-T), a rare neurodegenerative disease that causes multiple stress symptoms, including cerebellar degeneration, increased incidence of cancer, growth retardation, immune deficiencies, and premature aging (5). Cells derived from individuals with A-T are highly radiosensitive.
Central to the assay is the observation that ATM is activated in the cytoplasm and then translocates to the nucleus in order to activate the signaling that initiates in response to DNA DSBs (Figure 1). ATM’s functions in sensing DNA damage and signaling the onset of DNA repair and cell cycle checkpoint regulation would predict that this protein is localized to the nucleus. ATM has been widely observed in the nucleus (6). Importantly, however, ATM can also be found outside the nucleus including in various cytoplasmic organelles such as peroxisomes and endosomes. Indeed, it was reported that the localization of ATM to the peroxisome is activated by oxidative stress and it is delivered to this organelle via binding to the type 1 import receptor, peroxin 5 (PEX5) (7). Interestingly, this study also indicated that ATM localization to peroxisomes represses mammalian target of rapamycin complex 1 (mTORC1) signaling and induces autophagy. mTORC1 is known as a protein complex that activates translation. In addition to the peroxisome, the other vesicular organelle that ATM has been found to reside in is the endosome (8). It has been shown that the amino terminal domain of ATM interacts directly with ß-adaptin, one of the components of AP-2 adaptor complex that is involved in the clathrin-mediated receptor pathway. The interaction between ATM and ß-adaptin was confirmed in vivo by co-immunoprecipitation and immunofluorescence experiments suggesting that ATM may have physiological functions in the endocytic pathway. One possibility, then, is that there is a competition between cytoplasmic and nuclear functions of ATM and that this balance is altered towards the cytoplasmic functions in radiosensitive patients.
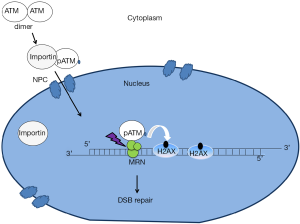
ATM nucleo-shuttling and DNA damage response
While cytoplasmic ATM functions have been described, the other key feature of the mechanism proposed by Granzotto et al. (2) is that ATM shuttles between the nucleus and the cytoplasm and, that in response to DSBs, ATM will be recruited from the cytoplasm to the nucleus. What is the evidence for nucleocytoplasmic shuttling? Several studies indicate that radiation-induced DSBs lead to the oxidation of ATM dimers that triggers ATM auto-phosphorylation on S1981 (pATM), monomerization in the cytoplasm and subsequent nuclear import (8-10). In the current study, the authors used immunofluorescence of fixed cells and confirmed this result by immunoblotting of cytoplasmic and nuclear fractions using a pATM antibody. This provides evidence that the phosphorylated forms of ATM are abundant and cytoplasmic (9). The two concerns are: (I) that the immunofluorescence reflects antibody binding to proteins in addition to ATM and (II) that soluble nuclear proteins can leak out of the nucleus during nuclear isolation. These caveats are difficult to circumvent experimentally and add a degree of doubt with respect to the proposed underlying biology responsible for the effectiveness of the assay.
Like many DNA repair proteins, ATM contains several nuclear localization signals (NLSs), which may be essential for its nuclear localization. In addition, ATM is a 370 kDa protein and its nuclear import may be similar to most nuclear proteins larger than 40–60 kDa, which require active transport to enter the nucleus. This active transport is facilitated by nuclear transport receptors, importins, which recognize nuclear localization sequences. Subsequently, the complex of a cargo protein (pATM) and a nuclear transport receptor is then shuttled from the cytoplasm into the nucleus through the nuclear pore complexes (NPC) by forming transient interactions with nucleoporins that line the channel of the pore (11). In the absence of DSBs, ATM kinase may dissociate from importins and diffuse in the nucleus without forming visible foci. However, after radiation-induced damage, ATM kinase localizes to DSB sites through an interaction with the C-terminus of the NBS1 component of the Mre11-Rad50-NBS1 (MRN) complex (12). Since MRN directly associates with DSB ends, this allows ATM recruitment to be upstream in the signaling pathway. Evidence that ATM executes some of its signaling functions through the MRN complex was revealed by studies of radiation sensitivity caused by mutation of either Mre11 or Nbs1. Mutations in Mre11 cause the A-T like disorder (ATLD), and mutations in the NBS1 gene have been found to cause Nijmegen breakage syndrome (NBS). ATLD and NBS share some clinical features with A-T such as radiation sensitivity, chromosomal instability and predisposition to cancer (13).
In the course of DSB repair, ATM phosphorylates serine 139 of histone H2AX adjacent to the site of damage, to generate the chromatin mark, γ-H2AX. γ-H2AX foci are used to detect the number of DSBs per cell as there is a one-to-one correlation between DSBs and γ-H2AX nuclear foci (14). In addition to ATM, DNA-PKcs (a kinase that is recruited to DSBs via an interaction with KU70-KU80 complex), is also involved in the phosphorylation of histone H2AX (15). However, an analysis of γ-H2AX foci kinetics in DNA-PKcs-/- cells showed that ATM is the essential kinase that phosphorylates γ-H2AX at least in the first hour after irradiation (16). Moreover, ATM also phosphorylates other DNA damage response proteins such as Mediator of DNA damage Checkpoint protein 1 (MDC1). MDC1 is recruited to damage sites through its interaction with γ-H2AX and in turn promotes the retention of ATM at the damage sites. Mre11, γ-H2AX and MDC1 foci arise in the first seconds to minutes post-damage. Subsequently, DSBs are repaired mainly by non-homologous end joining (NHEJ) or homologous recombination (HR) (17).
Shuttling of ATM into the nucleus thus appears to play critical roles in DSB recognition and repair. This concept is reinforced by the observation that decreasing ATM nuclear import in some genetic syndromes such as Huntington’s disease (HD) may result in a delay in the DSB recognition step and significant radiosensitivity. HD is caused by mutations of the huntingtin (HTT) gene. Ferlazzo et al. suggested that the functional form of huntingtin protein in the cytoplasm is required for normal ATM kinase activity in the nucleus, but the mutated huntingtin form sequesters ATM in the cytoplasm and limits DSB recognition and repair in the nucleus (18).
In addition to its nuclear import, ATM can also be exported from the nucleus in a mechanism first described by Wu et al. (19). In response to a DSB and ATM activation, ATM phosphorylates NEMO, which serves as a signal for mono-ubiquitylation of NEMO. This modification allows NEMO and ATM to translocate out of the nucleus where NEMO acts as an adaptor protein to bring together regulatory subunits of IκB. This activates the signaling cascade that allows activated NF-κB to re-enter the nucleus to transcriptionally regulate anti-apoptotic genes.
Regulation of ATM nucleo-shuttling
The data mentioned above indicates that ATM can be shuttled between nucleus and cytoplasm. However, it is not clear whether this shuttling can be regulated. One study has shown that there are distinct cytoplasmic pools of ATM that are activated in the cytoplasm and not localized to the nucleus (20). Indeed, H2O2-induced damage leads to cytoplasmic and nuclear ATM activation in MCF7 cells and treatment of those cells with leptomycin B, a potent inhibitor of the nuclear export receptor CRM1/exportin1, does not prevent the activation of ATM in the cytoplasm. Interestingly, this study shows that the substrates of ATM cytoplasmic pools display exclusively cytoplasmic localization. The study also suggested that the localization of ATM’s phosphorylated substrates might regulate ATM nucleo-cytoplasmic shuttling.
In addition to ATM, the MRN complex also displays nucleocytoplasmic shuttling, which is involved in DSB recognition and DNA repair. Desai-Mehta et al. demonstrated that NBS1 binds to Mre11 and Rad50 in the cytoplasm and directs the nuclear localization of the MRN complex, as well as formation of radiation-induced foci (21). NBS1 contains both NLS and a Nuclear Export Signal (NES) sequences required for nuclear-cytoplasmic shuttling of the MRN complex (22). While the need for nuclear import of NBS1 and the other members of the MRN complex is clear, the role of nuclear export in the function of the MRN complex is not understood. Like ATM, it might be possible that the MRN complex also displays some cytoplasmic functions which are separate from its role in the DNA damage response. In this regard, it has been reported that overexpression of human NBS1 can result in activation of phosphatidylinositol (PI) 3-kinase. Importantly, this activation may involve interaction between NBS1 and subunits of the PI 3-kinase, which would be expected to occur in the cytoplasm (23). The MRN complex could alter ATM accumulation in nuclear foci in two ways: (I) it might co-translocate ATM into the cytoplasm and (II) it might be required to bind activated ATM in the nucleus to prevent nuclear export. Since the MRN complex is also upstream of histone H2AX phosphorylation, it may be that measuring the subcellular distribution of NBS1 is sufficient to predict radiation sensitivity. Exploring this avenue might be fruitful if the underlying mechanism can be confirmed.
Concluding remarks
When using antibodies, we always have to consider the possibility that what is being recognized by the antibody is not the intended target. In the case of the antibody directed against phosphorylated ATM, we must consider that this phosphorylated region of ATM shares similarities to other substrates and that there is the potential for cross-reactivity. It is therefore important to understand mechanisms if we want to build on and improve the assay for radiation response assay for predicting radiation sensitivity. Given the challenges and expense that will likely be associated with the clinical implementation of the assay developed by Granzotto et al. (2) there is obvious value in pursuing a better understanding of the mechanism. Nonetheless, the assay clearly has utility as designed and it does correlate well with patient radiosensitivity. In that sense, the reagents and approach represent an advance regardless of the underlying biology.
Acknowledgments
Funding: F Ouenzar is supported by a Cancer Research Society (Canada) grant to MJ Hendzel.
Footnote
Provenance and Peer Review: This article was commissioned and reviewed by the Section Editor Hongcheng Zhu (Department of Radiation Oncology, The First Affiliated Hospital of Nanjing Medical University, Nanjing, China).
Conflicts of Interest: All authors have completed the ICMJE uniform disclosure form (available at http://dx.doi.org/10.21037/tcr.2016.10.61). The authors have no conflicts of interest to declare.
Ethical Statement: The authors are accountable for all aspects of the work in ensuring that questions related to the accuracy or integrity of any part of the work are appropriately investigated and resolved.
Open Access Statement: This is an Open Access article distributed in accordance with the Creative Commons Attribution-NonCommercial-NoDerivs 4.0 International License (CC BY-NC-ND 4.0), which permits the non-commercial replication and distribution of the article with the strict proviso that no changes or edits are made and the original work is properly cited (including links to both the formal publication through the relevant DOI and the license). See: https://creativecommons.org/licenses/by-nc-nd/4.0/.
References
- Chua ML, Rothkamm K. Biomarkers of radiation exposure: can they predict normal tissue radiosensitivity? Clin Oncol (R Coll Radiol) 2013;25:610-6. [Crossref] [PubMed]
- COPERNIC project investigators. Influence of Nucleoshuttling of the ATM Protein in the Healthy Tissues Response to Radiation Therapy: Toward a Molecular Classification of Human Radiosensitivity. Int J Radiat Oncol Biol Phys 2016;94:450-60. [Crossref] [PubMed]
- Shiloh Y. ATM and related protein kinases: safeguarding genome integrity. Nat Rev Cancer 2003;3:155-68. [Crossref] [PubMed]
- Bhatti S, Kozlov S, Farooqi AA, et al. ATM protein kinase: the linchpin of cellular defenses to stress. Cell Mol Life Sci 2011;68:2977-3006. [Crossref] [PubMed]
- Rotman G, Shiloh Y. ATM: from gene to function. Hum Mol Genet 1998;7:1555-63. [Crossref] [PubMed]
- Lavin MF. Ataxia-telangiectasia: from a rare disorder to a paradigm for cell signalling and cancer. Nat Rev Mol Cell Biol 2008;9:759-69. [Crossref] [PubMed]
- Zhang J, Tripathi DN, Jing J, et al. ATM functions at the peroxisome to induce pexophagy in response to ROS. Nat Cell Biol 2015;17:1259-69. [Crossref] [PubMed]
- Lim DS, Kirsch DG, Canman CE, et al. ATM binds to beta-adaptin in cytoplasmic vesicles. Proc Natl Acad Sci U S A 1998;95:10146-51. [Crossref] [PubMed]
- Bodgi L, Foray N. The nucleo-shuttling of the ATM protein as a basis for a novel theory of radiation response: resolution of the linear-quadratic model. Int J Radiat Biol 2016;92:117-31. [Crossref] [PubMed]
- Yang DQ, Halaby MJ, Li Y, et al. Cytoplasmic ATM protein kinase: an emerging therapeutic target for diabetes, cancer and neuronal degeneration. Drug Discov Today 2011;16:332-8. [Crossref] [PubMed]
- Görlich D, Mattaj IW. Nucleocytoplasmic transport. Science 1996;271:1513-8. [Crossref] [PubMed]
- You Z, Chahwan C, Bailis J, et al. ATM activation and its recruitment to damaged DNA require binding to the C terminus of Nbs1. Mol Cell Biol 2005;25:5363-79. [Crossref] [PubMed]
- Carney JP, Maser RS, Olivares H, et al. The hMre11/hRad50 protein complex and Nijmegen breakage syndrome: linkage of double-strand break repair to the cellular DNA damage response. Cell 1998;93:477-86. [Crossref] [PubMed]
- Rothkamm K, Löbrich M. Evidence for a lack of DNA double-strand break repair in human cells exposed to very low x-ray doses. Proc Natl Acad Sci U S A 2003;100:5057-62. [Crossref] [PubMed]
- Stiff T, O'Driscoll M, Rief N, et al. ATM and DNA-PK function redundantly to phosphorylate H2AX after exposure to ionizing radiation. Cancer Res 2004;64:2390-6. [Crossref] [PubMed]
- Joubert A, Zimmerman KM, Bencokova Z, et al. DNA double-strand break repair defects in syndromes associated with acute radiation response: at least two different assays to predict intrinsic radiosensitivity? Int J Radiat Biol 2008;84:107-25. [Crossref] [PubMed]
- Takata M, Sasaki MS, Sonoda E, et al. Homologous recombination and non-homologous end-joining pathways of DNA double-strand break repair have overlapping roles in the maintenance of chromosomal integrity in vertebrate cells. EMBO J 1998;17:5497-508. [Crossref] [PubMed]
- Ferlazzo ML, Sonzogni L, Granzotto A, et al. Mutations of the Huntington's disease protein impact on the ATM-dependent signaling and repair pathways of the radiation-induced DNA double-strand breaks: corrective effect of statins and bisphosphonates. Mol Neurobiol 2014;49:1200-11. [Crossref] [PubMed]
- Wu ZH, Shi Y, Tibbetts RS, et al. Molecular linkage between the kinase ATM and NF-kappaB signaling in response to genotoxic stimuli. Science 2006;311:1141-6. [Crossref] [PubMed]
- Alexander A, Cai SL, Kim J, et al. ATM signals to TSC2 in the cytoplasm to regulate mTORC1 in response to ROS. Proc Natl Acad Sci U S A 2010;107:4153-8. [Crossref] [PubMed]
- Desai-Mehta A, Cerosaletti KM, Concannon P. Distinct functional domains of nibrin mediate Mre11 binding, focus formation, and nuclear localization. Mol Cell Biol 2001;21:2184-91. [Crossref] [PubMed]
- Vissinga CS, Yeo TC, Warren S, et al. Nuclear export of NBN is required for normal cellular responses to radiation. Mol Cell Biol 2009;29:1000-6. [Crossref] [PubMed]
- Chen YC, Chiang HY, Yang MH, et al. Activation of phosphoinositide 3-kinase by the NBS1 DNA repair protein through a novel activation motif. J Mol Med (Berl) 2008;86:401-12. [Crossref] [PubMed]