Establishment and application of bladder cancer patient-derived xenografts as a novel preclinical platform
Introduction
Unresectable or metastatic urothelial carcinoma is best treated with systemic cisplatin-based chemotherapy. However, few patients are able to achieve durable disease control and often experience drug resistance and eventually death (1). The survival of patients with metastatic urothelial carcinoma has not markedly improved for more than 20 years (2), in part because of the paucity of disease models that recapitulate clinical behavior such as the drug response of urothelial carcinoma.
The successful development of effective anticancer drugs requires a preclinical model system that accurately predicts the safety and efficacy of promising compounds in clinical trials. The use of xenograft models implanted with human cancer cell lines has remained the standard practice for preclinical drug evaluation for the past 30 years. However, most de novo anti-cancer drugs fail to reach even phase III clinical trials. The discrepancy between preclinical promise and clinical reality raises concerns regarding the effectiveness of the traditional subcutaneous xenograft model (3). The lack of preclinical models that preserve the heterogeneity of the primary tumor, and the poor biologic and genetic reproducibility and low predictive value of existing models have been major hurdles in cancer drug development (4,5).
To circumvent the limitations of the traditional subcutaneous xenograft model, the use of patient-derived xenografts (PDXs) has emerged as an alternative preclinical model and has been studied actively to explore their potential. PDX models are now being consistently characterized and applied in oncology research.
PDX models are characterized by direct engraftment of patient-derived tumor fragments into immunocompromised mice. These models have proven to be more representative of human patients compared with other xenograft models or in vitro models. Several studies performed in the 1980s investigated the validity of PDX models by comparing the response to chemotherapy in mouse models, and most of them found a high degree of correlation in the response to chemotherapy between patients and the corresponding PDX models (6-8). PDX models have been developed in various organ systems, including brain cancer, prostate cancer, pancreatic cancer, breast cancer, ovarian and gynecologic cancer, colorectal cancer, lung cancer, hematologic disorders, and melanoma (4,5,9-15).
The use of PDX models to select appropriate treatments and discover new, more effective, and safer drugs is expected to contribute to reducing morbidity and costs associated with urologic cancer, which is increasing in prevalence. In this review, we discuss the advantages and disadvantages of PDX models in drug development, their application in bladder cancer fields, and the future direction of PDX models.
Cancer cell line xenograft models
Traditionally, cancer cell lines derived from patient tumors have been widely used in cancer research. By the early 1970s, multiple human tumors had been cultured in vitro, leading to collections of human tumor cell lines. The primary advantage of cell line-based xenograft models in mice compared to in vitro models is to recapitulate the complex microenvironment, which allows interaction between cancer cells and the host vasculature and stroma. These models are simple, consistent, and cost effective as well.
However, cell line-based xenograft models have several unavoidable limitations. First, cell line-based xenografts tend to lose the heterogeneity and many critical genetic signatures of the original tumor. The process of cell culturing results in a phenotypically homogeneous culture, because the resident cells and proteins of the original tumor are eliminated, and only tumor cells that are favorable to the culture environment survive and are selected (5). Thus, the successfully grown tumor differs from the original heterogeneous source. Cancer cell line xenografts are not representative of the drug response or resistance shown by primary tumors (16). Johnson et al. evaluated potential anti-cancer agents in xenograft studies and phase II trials and noted that the correlation between the results of xenograft studies and phase II trials was very limited (3).
Retrospective studies have shown that many compounds with promising activity in xenograft models did not lead to clinical success owing to limited efficacy or high toxicity (17). The FDA approves only about 5% of anti-cancer agents after pre-clinical testing in the absence of a human stromal environment and limited tumor heterogeneity (16).
Advantages and disadvantages of PDX in drug development
Preclinical models that are able to predict the efficacy and safety of test compounds are required for the successful development of anti-cancer agents. PDX has attracted attention as an alternative preclinical model for cancer research to overcome the limitations of cancer cell lines. Thus, PDX models have been applied to various fields of cancer research including tumor biology, personalized cancer therapy, cancer drug screening, biomarker discovery, and investigating drug resistance mechanisms, as summarized below (18).
First, PDX models are appropriate tools for expanding knowledge of tumor biology on the basis of the vast majority of reports that describe histological similarity between the original tumor and PDX. In terms of genetic fidelity, the parental tumor and PDX generally share most (>90%) genetic alterations in urothelial carcinoma (19). The histological characteristics of the original tumor are preserved for three to six passages (19,20). Potential therapeutic targets in bladder cancer PDX have been identified using fluorescence-activated cell sorting (FACS) techniques. The combined use of FACS and PDX models resulted in successful molecular profiling and identification of a gene signature of bladder tumor-initiating cells that was associated with poor prognosis (21). Those studies also highlighted the roles of cancer stem cells in therapeutic resistance in bladder cancer using PDXs, finding that CK14+ cells contribute to tumor regrowth by activating a proliferation response after chemotherapy-induced damage, and these cells demonstrated the necessary functional criteria to be considered cancer stem cells (21).
Second, PDX models are believed to be an ideal tool for personalized precision medicine (22), although their clinical value for urological malignancies has not been fully established yet. Using PDX, a pilot test to evaluate therapeutic responses to anti-cancer agents can be performed before applying them to patients. Stebbing et al. successfully established 22 sarcoma PDX models from 29 patients and screened them for a panel of chemotherapeutic agents, with a 76% take rate during a period of 3−6 months (23). Six patients died before test data were available. A correlation between prediction from PDX evaluations and clinical outcome was observed in 81% of the tested cases (18). This process not only provides an opportunity for patients to be treated with appropriate anti-cancer agents, but also has the effect of sparing them from exposure to a drug that may have no effect (24).
Third, PDX models are widely used as screening platforms for clinical drug trials. Advances in experimental methods have revealed that tumor heterogeneity is a hallmark of cancer and so is, bladder cancer (25). Since PDX avoids the use of an in vitro process, PDX models are expected to recapitulate the complexity of human cancer, with preserved tumor heterogeneity, cellular lineage hierarchy, and tumor-stroma interaction. Indeed, a high correlation in drug response between PDX models and patients has been reported (22,26).
Finally, PDX models also enable the discovery of biomarkers to predict oncologic drug sensitivity and resistance. PDX models from colorectal cancer patients revealed that KRAS mutant tumors did not respond to the anti-epidermal growth factor receptor antibody cetuximab (27-29). KRAS wild-type status is now a well-documented clinical biomarker for this targeted therapy. An extensive analysis of cetuximab in 47 colorectal cancer PDX models showed a 10.6% response rate, consistent with the response rate observed in patients with this disease (29). Detailed analysis of the mechanisms underlying resistance to EGFR inhibitors in these PDX models resulted in the identification of HER2 and MET amplification as predictors of resistance, leading to novel clinical trials (29,30).
One of the drawbacks of PDX models is the inevitable requirement of immunodeficient mice to prevent immune attacks against the xenografted tumor. The use of immunodeficient mice as PDX hosts has several limitations. First, the complex microenvironments of human primary tumors are not recapitulated in immunodeficient mice. Despite the successful growth of primary human tumors in immunodeficient mice, the microenvironment including tumor-promoting cells is replaced by a murine equivalent. In this case, drugs that target human cells may not function appropriately. Validation studies of PDX models found a significant stromal loss of tumor after multiple murine transplantations (8,31). Another limitation of immunodeficient mice is that graft-versus-host and anticancer immunity may be not fully interrupted during the PDX process, resulting in engraftment failure (32). Furthermore, lymphangiogenesis and angiogenesis promoted by B lymphocytes and macrophages may influence tumor growth and metastasis (33,34). Once a requisit for microenvironment interaction of tumor is disappeared, which result in unavailability of evaluating anti-cancer drugs that has effect on immune system or immunotherapies.
Xenotransplantation of human cancer tissue into immunocompromised mice has been reported to occasionally induce synergetic tumors that are completely different from the parental tumors. Mouse spindle cell tumors can occur at the transplantation site of host animals, suggesting that tumor cells might trigger the transformation of neighboring stromal cells into sarcomatoid tumors through humoral factors expressed by the tumor cells, although this phenomenon is rare (35,36).
Low engraftment rates and the long latency time for establishing the models are also pitfalls of PDX. Furthermore, the requirement for a relatively long period from setting up an ‘Avatar model’ to actual application of the appropriate anti-cancer agent is another inevitable hurdle. In prior case series, the time needed to establish xenografts and test drugs usually exceeded the patients’ life span (8). In addition, some tumors are associated with infections by bacteria, viruses, and parasites (37). PDX models using SCID-based mouse strains have demonstrated more successful engraftment rates than nude mouse-based strains. However, NOD-SCID mice only live up to 37 weeks and can develop thymic lymphoma, which makes it impossible to observe the long-term effects and safety of therapeutic treatments in models employing these mice (38,39). Finally, the high cost of establishing PDX models is another hurdle to starting and maintaining them.
Application of PDX models in bladder cancer
Tumor extraction and take rate
Cancer tissues can be extracted from patients after obtaining informed consent. Fresh tumor tissue from surgical or tumor biopsy specimens determines the successful establishment of PDXs in mice. Bladder cancer is particularly suitable for the development of PDX models because bladder cancers are large, and fresh tissue can easily be obtained when the tumors are resected transurethrally. In other words, surgery can provide abundant material with a short latency time to inoculation into the mouse. In a prior study, a total of 65 human bladder cancer tissue samples were implanted into the flank subcutaneous layer of immunodeficient mice (40). Among these 65 cases, six PDX models were successfully generated, and primary tumor tissues and xenografts of passage ≥2 or higher were compared. After hematoxylin and eosin staining and array-comparative genomic hybridization (CGH) analysis, similar histologic characteristics, degree of differentiation, and genomic alterations were observed in the primary and xenograft tumors. Advanced stage and increased pathological grade have been associated with improved tumor take rate for urothelial carcinoma PDX (41).
Several other previous studies reported the outcomes of bladder cancer PDX models. Reported tumor take rates range from 11% to 80% (20,42-47). There are differences in details concerning host mouse, site of tumor implantation, tumor histology, tumor sample, whether Matrigel was used or not, and era of PDX model development. Previously reported bladder cancer PDX models are summarized in Table 1.
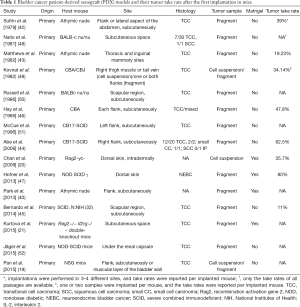
Full table
Selection of host mouse strains
Several types of immunocompromised mice are available for xenograft transplantation. Athymic (nude) mice are most commonly used for the establishment of PDX mouse models, which harbor a Foxn1 gene mutation, that results in the absence of mature T cells (53,54). SCID (55) and Rag1- or Rag2-null (56,57) are adaptive immune compromised mouse strains.
Several severe combined immunodeficient mouse strains have been described, including SCID beige (58); nonobese diabetic (NOD)-SCID (59); NOD-SCID-gamma (58,60,61); NOG (62,63); Rag-gamma and Balb-Rag-gamma (BRG) (64); NOD-Rag-gamma (NRG) (65); NOD-SCID-gamma HLA-A2.1 transgenic (37); and NOD-SCID-gamma IL-3, GM-CSF, and SCF (NSG-SGM3) transgenic (66,67). NOD-SCID and NOD-Rag strains demonstrated better growth and survival of primary tumors, hematopoietic cells, and hematologic malignancies.
Site of tumor tissue implantation
The most commonly used site for engraftment is the subcutaneous space on the dorsal side of mice (19,20,40,42,44-51). Subcutaneous xenografts are technically easy to handle, and monitoring and evaluating the tumor size are also simple.
In contrast to the subcutaneous graft site, the subrenal capsule site is highly vascularized and associated with a very high tumor take rate for urothelial cancer tissue. Jäger et al. reported five cases of bladder cancer PDX models, in which patient tumors were transplanted below the renal capsule of mice and grafted over multiple generations (52).
Orthotopic engraftment of fresh bladder cancer tissue might be advantageous, as it provides a more physiologically relevant environment for the growth of human bladder tissue. However, successful PDX establishment from orthotopic engraftment of urothelial tumor specimens has not been achieved, although the submucosal injection of dissociated cells could be technically viable (68,69).
Screening platforms for clinical drug trials
Hofner et al. generated patient-derived neuroendocrine bladder cancer xenograft models (47), noting that the expression of the hepatocyte growth factor (HGF) receptor MET was found in xenograft models, neuroendocrine bladder cancer cultures, and primary tumor sections. Neuroendocrine bladder cancer spheroid growth in vitro was dependent on HGF, and treatment with a MET inhibitor was associated with a significant decrease in tumor growth in PDX mice compared to control-treated mice, highlighting MET as a new treatment target for neuroendocrine bladder cancer. Furthermore, Kurtova et al. successfully developed a PDX model from urothelial carcinoma that recapitulated acquired chemoresistance over serial administrations of gemcitabine and cisplatin, which is the standard chemotherapy regimen for advanced urothelial carcinoma (21). They found that cytotoxic chemotherapy induced cyclooxygenase 2 (COX2)- and prostaglandin E2-mediated tumor repopulation, which contributed to the regrowth of chemoresistant tumors. A COX2 inhibitor effectively retarded the progressive manifestation of chemoresistance in PDX tumors (21).
Jäger et al. developed five PDX models of muscle invasive bladder cancer by implanting primary tumors under the renal capsule of NOD-SCID mice (52). Subsequently, the PDX and patient tumors were processed for analysis of gene expression with microarray, copy number variations with array CGH, and expression of target pathways with immunohistochemistry. One PDX harboring an FGFR3 mutation was treated with an inhibitory monoclonal antibody that was able to neutralize FGFR3. Shared chromosomal aberrations in the patient tumors and PDX were identified by array CGH. The parental tumors and PDXs demonstrated similar patterns on immunohistochemistry and gene expression microarray. The FGFR3 inhibitor inhibited tumor growth in the PDX that harbored an FGFR3 mutation.
Pan et al. established and characterized 22 PDX models from urothelial carcinoma tumors (19). Using whole-exome and RNA sequencing, they investigated the genetic characteristics of the PDXs and treatment response to an epidermal growth factor receptor–receptor tyrosine-protein kinase erbB-2 dual inhibitor, a phosphatidylinositol 4,5-bisphosphate 3-kinase catalytic subunit α isoform inhibitor, a proto-oncogene tyrosine-protein kinase Src inhibitor, and a fibroblast growth factor receptor 3 inhibitor. These authors observed good correlations between the genomic characteristics and treatment responses of PDX models and donor patients.
Future directions of PDX models
The ideal preclinical tumor model would be able to reproduce the biology of human cancer; to predict clinical response; and to be reliable, widely available, and affordable.
The establishment of PDX tumors requires the use of immunocompromised host strains. However, an increasing number of agents modulating the immune system have shown promise as therapies for bladder cancer (70). Therefore, many shortcomings of PDX in immunocompromised mice can be overcome by humanizing the immune system and the tumor microenvironment. Humanized mice are an attractive alternative for studying the contribution of the human immune system to tumor biology, facilitating the assessment of the tumor microenvironment, and evaluating the efficacy of immunomodulatory agents. Humanized mice can be created by engrafting human hematopoietic stem cells into immunocompromised mice, giving rise to a functional human immune system (71-73).
Humanizing the tumor microenvironment is also important for improving PDX mouse models. Targeted genome editing technologies such as zinc finger nucleases, transcription activator-like effector nucleases, and CRISPR/Cas9 have been advanced and used in the development of improved NSG mice, which have additional innate defects and express human cytokines (74). As additional growth requirements for primary patient-derived tumors and stromal/immune cells are noted, the development of new models will continue, thus enabling the use of more predictive PDX tumor models to assess therapeutic response and patient survival.
In practice, the failure of certain PDX tumors to engraft is still high. For application in personalized medicine, the tumor take rates must be improved to 60–70%, which is one of the main issues that needs addressing. Approaches to improving modeling in PDX mice include further decreasing anti-tumor immunity. For instance, TLR signaling may accord antitumor immunity. Production of NSG mice with innate immune defects, such as Myd88–/– or utilization of MISTRG mice that also express human SIRPα and lack alveolar macrophages could promote tumor engraftment (14,19).
The generation and propagation of PDX tumors and subsequent drug testing typically take 4–6 months, which is usually not a feasible timeframe for immediate application in clinical decision-making for personalized therapeutics. Accordingly, methods for reducing the time needed for engraftment are necessary for the realization of personalized medicine with PDX models. Recently, cancer organoid models have emerged as a method for preclinical models and have showed promising results with regard to their shorter generation and propagation time and a higher success rate compared with that seen in PDX models.
Advances in cancer organoid models
The term ‘organoid’ has historically been used to encompass all three-dimensional organotypic cultures derived from primary tissues, embryonic stem cells, and induced pluripotent stem cells, as well as whole or segmented organs such as organ explants consisting of multiple tissue types (75). These organoids rely on artificial extracellular matrices to facilitate their self-organization into structures that resemble native tissue architecture, are capable of self-renewal and self-organization, and exhibit similar organ functionality as that of the tissue of origin.
Recently, the organoid cancer model has been gaining attention as a promising preclinical model for the development of new anti-cancer therapeutics. Efforts to establish a large repertoire of in vitro organoid cancer models that can represent the heterogeneity of human cancers and provide a resource for cancer biology research and drug response screening are in progress. Sato et al. reported the building of crypt-villus structures using single Lgr5 stem cells without a mesenchymal niche in vitro (76). An optimized culture system with laminin-rich Matrigel and signaling and growth factors was developed for the growth of intestinal structures with crypt-villus architecture that is comparable to intestinal epithelium in vivo (76). Several cell types generated from organ progenitors or stem cells are contained in organoids. Prior studies have reported organoid models of benign and malignant prostate, colon, liver, pancreas, stomach, and thyroid (77-81).
In previous studies, various efforts have been reported in the field of bladder organoids. Leighton et al. reported small-scale mucosal models of rats (82), and Huygens et al. established spheroids (83). A model of human bladder cancer invasion (84) and recurrence in vitro (85) were reported using rat and porcine bladders, respectively. Smith et al. reported a bladder benign organoid model with human 5637 (HTB-9) cells that showed in vivo tissue-specific differentiation (86). An experimental ex vivo organoid model of the bladder mucosa for preclinical experimental research that can serve as an alternative to animal models was developed by Janssen et al. (87). Cells proliferated, and they were noted in smooth muscle cell medium and Dulbecco’s modified Eagle’s medium/F-12 for up to 3 weeks, and morphological characteristics of the mucosa retained normal characteristics for up to 1 week. Palmer et al. developed a bladder muscle invasive transitional cell carcinoma model using the human bladder cancer cell line 5637 and porcine bladder scaffold (88).
Cancer organoid models have advantages of cancer cell lines and PDX models with regard to the feasibility of manipulation and drug screening, and more physiologic characteristics, respectively (89). Adult stem cells can multiply in organoids, and specific tissue lines with a high purity can be cultured. Organoids can propagate for a long period of time without genomic modification; can be applied to a variety of established methods; and can be derived from multiple sources, such as fetal and adult tissues, induced pluripotent stem cells, and traditional embryonic stem cells. Organoids that contain a wide range of tissues can be produced by organoid culture. Additionally, organoid models enable a limited amount of starting materials to be used for a variety of applications. The generation of isogenic adult tissue for transplantation may be possible as well. In addition, diseases that are challenging to model in animals can be investigated using patient-derived organoids.
However, the generation of large numbers of organoid lines is required to cover the spectrum of alterations of bladder cancer for successful drug screening. In addition, to better recapitulate the in vivo environment and improve the efficiency of establishment, the continued refinement of culturing conditions is needed. Organoid modeling is a promising candidate for personalized medicine; however, prior representation of patient response by in vitro response with bladder cancer organoid cultures must be established in order for them to serve as a preclinical model for therapy selection.
Summary
PDX models retain the histology, genomic characteristics, and drug responsiveness of the corresponding patient tumor. Despite several limitations that must be resolved, the use of PDX models is expected to render further understanding of tumor characteristics and to contribute to improving the treatment of cancer patients. Recently, a single-arm phase II trial indicated that treatment with the anti-programmed cell death 1 ligand antibody atezolizumab is a potential treatment approach for patients with locally advanced or metastatic urothelial carcinoma who are not eligible to receive cisplatin-based chemotherapy (90). Thus, establishing effective protocols for the generation of urothelial carcinoma PDXs in humanized mice is urgently needed.
Acknowledgments
Funding: This work was supported by grants (NCC1610230) from the National Cancer Center of the Republic of Korea.
Footnote
Provenance and Peer Review: This article was commissioned by the Guest Editors (Ja Hyeon Ku, Kunyoo Shin, Minyong Kang) for the series “Bladder Cancer” published in Translational Cancer Research. The article has undergone external peer review.
Conflicts of Interest: All authors have completed the ICMJE uniform disclosure form (available at http://dx.doi.org/10.21037/tcr.2017.06.27). The series “Bladder Cancer” was commissioned by the editorial office without any funding or sponsorship. The authors have no other conflicts of interest to declare.
Ethical Statement: The authors are accountable for all aspects of the work in ensuring that questions related to the accuracy or integrity of any part of the work are appropriately investigated and resolved.
Open Access Statement: This is an Open Access article distributed in accordance with the Creative Commons Attribution-NonCommercial-NoDerivs 4.0 International License (CC BY-NC-ND 4.0), which permits the non-commercial replication and distribution of the article with the strict proviso that no changes or edits are made and the original work is properly cited (including links to both the formal publication through the relevant DOI and the license). See: https://creativecommons.org/licenses/by-nc-nd/4.0/.
References
- Kamat AM, Hahn NM, Efstathiou JA, et al. Bladder cancer. Lancet 2016;388:2796-810. [Crossref] [PubMed]
- Prasad SM, DeCastro GJ, Steinberg GD. Urothelial carcinoma of the bladder: definition, treatment and future efforts. Nat Rev Urol 2011;8:631-42. [Crossref] [PubMed]
- Johnson JI, Decker S, Zaharevitz D, et al. Relationships between drug activity in NCI preclinical in vitro and in vivo models and early clinical trials. Br J Cancer 2001;84:1424. [Crossref] [PubMed]
- DeRose YS, Wang G, Lin YC, et al. Tumor grafts derived from women with breast cancer authentically reflect tumor pathology, growth, metastasis and disease outcomes. Nat Med 2011;17:1514-20. [Crossref] [PubMed]
- Tentler JJ, Tan AC, Weekes CD, et al. Patient-derived tumour xenografts as models for oncology drug development. Nat Rev Clin Oncol 2012;9:338-50. [Crossref] [PubMed]
- Fiebig HH, Schuchhardt C, Henss H, et al. Comparison of tumor response in nude mice and in the patients. Behring Inst Mitt 1984;74:343-52. [PubMed]
- Osieka R. Human malignant melanoma: preclinical and clinical drug evaluation. Behring Inst Mitt 1984;353-67. [PubMed]
- Shorthouse AJ, Smyth J, Steel G, et al. The human tumour xenograft--a valid model in experimental chemotherapy? Br J Surg 1980;67:715-22. [Crossref] [PubMed]
- Braekeveldt N, Wigerup C, Gisselsson D, et al. Neuroblastoma patient-derived orthotopic xenografts retain metastatic patterns and geno- and phenotypes of patient tumours. Int J Cancer 2015;136:E252-61. [Crossref] [PubMed]
- Laheru D, Shah P, Rajeshkumar N, et al. Integrated preclinical and clinical development of S-trans, trans-Farnesylthiosalicylic Acid (FTS, Salirasib) in pancreatic cancer. Invest New Drugs 2012;30:2391-9. [Crossref] [PubMed]
- Hidalgo M, Bruckheimer E, Rajeshkumar N, et al. A pilot clinical study of treatment guided by personalized tumorgrafts in patients with advanced cancer. Mol Cancer Ther 2011;10:1311-6. [Crossref] [PubMed]
- Krumbach R, Schüler J, Hofmann M, et al. Primary resistance to cetuximab in a panel of patient-derived tumour xenograft models: activation of MET as one mechanism for drug resistance. Eur J Cancer 2011;47:1231-43. [Crossref] [PubMed]
- De Sousa E Melo F, Wang X, Jansen M, et al. Poor-prognosis colon cancer is defined by a molecularly distinct subtype and develops from serrated precursor lesions. Nat Med 2013;19:614-8. [Crossref] [PubMed]
- Guenot D, Guerin E, Aguillon-Romain S, et al. Primary tumour genetic alterations and intra-tumoral heterogeneity are maintained in xenografts of human colon cancers showing chromosome instability. J Pathol 2006;208:643-52. [Crossref] [PubMed]
- Romanelli A, Clark A, Assayag F, et al. Inhibiting aurora kinases reduces tumor growth and suppresses tumor recurrence after chemotherapy in patient-derived triple-negative breast cancer xenografts. Mol Cancer Ther 2012;11:2693-703. [Crossref] [PubMed]
- Hutchinson L, Kirk R. High drug attrition rates—where are we going wrong? Nat Rev Clin Oncol 2011;8:189-90. [Crossref] [PubMed]
- Suggitt M, Bibby MC. 50 years of preclinical anticancer drug screening: empirical to target-driven approaches. Clin Cancer Res 2005;11:971-81. [PubMed]
- Malaney P, Nicosia SV, Davé V. One mouse, one patient paradigm: New avatars of personalized cancer therapy. Cancer Lett 2014;344:1-12. [Crossref] [PubMed]
- Pan CX, Zhang H, Tepper CG, et al. Development and characterization of bladder cancer patient-derived xenografts for molecularly guided targeted therapy. PLoS One 2015;10:e0134346 [Crossref] [PubMed]
- Chan KS, Espinosa I, Chao M, et al. Identification, molecular characterization, clinical prognosis, and therapeutic targeting of human bladder tumor-initiating cells. Proc Natl Acad Sci U S A 2009;106:14016-21. [Crossref] [PubMed]
- Kurtova AV, Xiao J, Mo Q, et al. Blocking PGE2-induced tumour repopulation abrogates bladder cancer chemoresistance. Nature 2015;517:209-13. [Crossref] [PubMed]
- Gao H, Korn JM, Ferretti S, et al. High-throughput screening using patient-derived tumor xenografts to predict clinical trial drug response. Nat Med 2015;21:1318-25. [Crossref] [PubMed]
- Stebbing J, Paz K, Schwartz GK, et al. Patient-derived xenografts for individualized care in advanced sarcoma. Cancer 2014;120:2006-15. [Crossref] [PubMed]
- Richmond A, Su Y. Mouse xenograft models vs GEM models for human cancer therapeutics. Dis Model Mech 2008;1:78-82. [Crossref] [PubMed]
- Morrison CD, Liu P, Woloszynska-Read A, et al. Whole-genome sequencing identifies genomic heterogeneity at a nucleotide and chromosomal level in bladder cancer. Proc Natl Acad Sci U S A 2014;111:E672-81. [Crossref] [PubMed]
- Kerbel RS. Human tumor xenografts as predictive preclinical models for anticancer drug activity in humans: better than commonly perceived—but they can be improved. Cancer Biol Ther 2003;2:S134-9. [Crossref] [PubMed]
- Hidalgo M, Amant F, Biankin AV, et al. Patient-derived xenograft models: an emerging platform for translational cancer research. Cancer Discov 2014;4:998-1013. [Crossref] [PubMed]
- Julien S, Merino-Trigo A, Lacroix L, et al. Characterization of a large panel of patient-derived tumor xenografts representing the clinical heterogeneity of human colorectal cancer. Clin Cancer Res 2012;18:5314-28. [Crossref] [PubMed]
- Bertotti A, Migliardi G, Galimi F, et al. A molecularly annotated platform of patient-derived xenografts (“xenopatients”) identifies HER2 as an effective therapeutic target in cetuximab-resistant colorectal cancer. Cancer Discov 2011;1:508-23. [Crossref] [PubMed]
- Bardelli A, Corso S, Bertotti A, et al. Amplification of the MET receptor drives resistance to anti-EGFR therapies in colorectal cancer. Cancer Discov 2013;3:658-73. [Crossref] [PubMed]
- Shimosato Y, Kameya T, Nagai K, et al. Transplantation of human tumors in nude mice. J Natl Cancer Inst 1976;56:1251-60. [Crossref] [PubMed]
- Brehm MA, Cuthbert A, Yang C, et al. Parameters for establishing humanized mouse models to study human immunity: analysis of human hematopoietic stem cell engraftment in three immunodeficient strains of mice bearing the IL2rγ null mutation. Clin Immunol 2010;135:84-98. [Crossref] [PubMed]
- Ruddell A, Mezquita P, Brandvold KA, et al. B lymphocyte-specific c-Myc expression stimulates early and functional expansion of the vasculature and lymphatics during lymphomagenesis. Am J Pathol 2003;163:2233-45. [Crossref] [PubMed]
- Qian B-Z, Pollard JW. Macrophage diversity enhances tumor progression and metastasis. Cell 2010;141:39-51. [Crossref] [PubMed]
- Russell PJ, Brown J, Grimmond S, et al. Tumour-induced host stromal-cell transformation: Induction of mouse spindle-cell fibrosarcoma not mediated by gene transfer. Int J Cancer 1990;46:299-309. [Crossref] [PubMed]
- Goldenberg DM, Pavia RA. In vivo horizontal oncogenesis by a human tumor in nude mice. Proc Natl Acad Sci U S A 1982;79:2389-92. [Crossref] [PubMed]
- Chen K, Ahmed S, Adeyi O, et al. Human solid tumor xenografts in immunodeficient mice are vulnerable to lymphomagenesis associated with Epstein-Barr virus. PLoS One 2012;7:e39294 [Crossref] [PubMed]
- Landis MD, Lehmann BD, Pietenpol JA, et al. Patient-derived breast tumor xenografts facilitating personalized cancer therapy. Breast Cancer Res 2013;15:201. [Crossref] [PubMed]
- Shultz LD, Schweitzer PA, Christianson SW, et al. Multiple defects in innate and adaptive immunologic function in NOD/LtSz-scid mice. J Immunol 1995;154:180-91. [PubMed]
- Park B, Jeong BC, Choi YL, et al. Development and characterization of a bladder cancer xenograft model using patient-derived tumor tissue. Cancer Sci 2013;104:631-8. [Crossref] [PubMed]
- Kobayashi T, Owczarek TB, McKiernan JM, et al. Modelling bladder cancer in mice: opportunities and challenges. Nat Rev Cancer 2015;15:42-54. [Crossref] [PubMed]
- Sufrin G, McGarry M, Sandberg A, et al. Heterotransplantation of human transitional cell carcinoma in athymic mice. J Urol 1979;121:159-61. [PubMed]
- Matthews PN, Grant AG, Hermon-Taylor J. The growth of human bladder and kidney cancers as xenografts in nude mice and rats. Urol Res 1982;10:293-9. [Crossref] [PubMed]
- Abe T, Tada M, Shinohara N, et al. Establishment and characterization of human urothelial cancer xenografts in severe combined immunodeficient mice. Int J Urol 2006;13:47-57. [Crossref] [PubMed]
- Bernardo C, Costa C, Amaro T, et al. Patient-derived sialyl-Tn-positive invasive bladder cancer xenografts in nude mice: an exploratory model study. Anticancer Res 2014;34:735-44. [PubMed]
- Hay JH, Busuttil A, Steel C, et al. The growth and histological characteristics of a series of human bladder cancer xenografts. Radiother Oncol 1986;7:331-40. [Crossref] [PubMed]
- Hofner T, Macher-Goeppinger S, Klein C, et al. Development and characteristics of preclinical experimental models for the research of rare neuroendocrine bladder cancer. J Urol 2013;190:2263-70. [Crossref] [PubMed]
- Naito S, Iwakawa A, Tanaka K, et al. Heterotransplantation of human urinary bladder cancers in nude mice. Investigative urology 1981;18:285-8. [PubMed]
- Kovnat A, Armitage M, Tannock I. Xenografts of human bladder cancer in immune-deprived mice. Cancer Res 1982;42:3696-703. [PubMed]
- Russell PJ, Raghavan D, Gregory P, et al. Bladder cancer xenografts: a model of tumor cell heterogeneity. Cancer Res 1986;46:2035-40. [PubMed]
- McCue PA, Gomella LG, Veltri RW, et al. Development of secondary structure, growth characteristics and cytogenetic analysis of human transitional cell carcinoma xenografts in scid/scid mice. J Urol 1996;155:1128-32. [Crossref] [PubMed]
- Jäger W, Xue H, Hayashi T, et al. Patient-derived bladder cancer xenografts in the preclinical development of novel targeted therapies. Oncotarget 2015;6:21522. [Crossref] [PubMed]
- Day C-P, Merlino G, Van Dyke T. Preclinical mouse cancer models: a maze of opportunities and challenges. Cell 2015;163:39-53. [Crossref] [PubMed]
- Pantelouris EM. Absence of thymus in a mouse mutant. Nature 1968;217:370-1. [Crossref] [PubMed]
- Roychoudhuri R, Eil RL, Restifo NP. The interplay of effector and regulatory T cells in cancer. Curr Opin Immunol 2015;33:101-11. [Crossref] [PubMed]
- Bosma GC, Custer RP, Bosma MJ. A severe combined immunodeficiency mutation in the mouse. Nature 1983;301:527-30. [Crossref] [PubMed]
- Mombaerts P, Iacomini J, Johnson RS, et al. RAG-1-deficient mice have no mature B and T lymphocytes. Cell 1992;68:869-77. [Crossref] [PubMed]
- Shinkai Y, Koyasu S, Nakayama KI, et al. Restoration of T cell development in RAG-2-deficient mice by functional TCR transgenes. Science 1993;259:822-5. [Crossref] [PubMed]
- Wunderlich M, Chou FS, Link K, et al. AML xenograft efficiency is significantly improved in NOD/SCID-IL2RG mice constitutively expressing human SCF, GM-CSF and IL-3. Leukemia 2010;24:1785-8. [Crossref] [PubMed]
- Agliano A, Martin-Padura I, Mancuso P, et al. Human acute leukemia cells injected in NOD/LtSz-scid/IL-2Rγ null mice generate a faster and more efficient disease compared to other NOD/scid-related strains. Int J Cancer 2008;123:2222-7. [Crossref] [PubMed]
- Ishikawa F, Yasukawa M, Lyons B, et al. Development of functional human blood and immune systems in NOD/SCID/IL2 receptor γ chainnull mice. Blood 2005;106:1565-73. [Crossref] [PubMed]
- Shultz LD, Lyons BL, Burzenski LM, et al. Human lymphoid and myeloid cell development in NOD/LtSz-scid IL2Rγnull mice engrafted with mobilized human hemopoietic stem cells. J Immunol 2005;174:6477-89. [Crossref] [PubMed]
- Ito M, Hiramatsu H, Kobayashi K, et al. NOD/SCID/γ mouse: an excellent recipient mouse model for engraftment of human cells. Blood 2002;100:3175-82. [Crossref] [PubMed]
- Pearson T, Shultz LD, Miller D, et al. Non-obese diabetic–recombination activating gene-1 (NOD–Rag 1 null) interleukin (IL)-2 receptor common gamma chain (IL 2 rγnull) null mice: a radioresistant model for human lymphohaematopoietic engraftment. Clin Exp Immunol 2008;154:270-84. [Crossref] [PubMed]
- Yahata T, Ando K, Nakamura Y, et al. Functional human T lymphocyte development from cord blood CD34+ cells in nonobese diabetic/Shi-scid, IL-2 receptor γ null mice. J Immunol 2002;169:204-9. [Crossref] [PubMed]
- Nicolini FE, Cashman J, Hogge D, et al. NOD/SCID mice engineered to express human IL-3, GM-CSF and Steel factor constitutively mobilize engrafted human progenitors and compromise human stem cell regeneration. Leukemia 2004;18:341-7. [Crossref] [PubMed]
- Strowig T, Gurer C, Ploss A, et al. Priming of protective T cell responses against virus-induced tumors in mice with human immune system components. J Exp Med 2009;206:1423-34. [Crossref] [PubMed]
- Jäger W, Moskalev I, Janssen C, et al. Ultrasound-guided intramural inoculation of orthotopic bladder cancer xenografts: a novel high-precision approach. PLoS One 2013;8:e59536 [Crossref] [PubMed]
- Jäger W, Moskalev I, Janssen C, et al. Minimally invasive establishment of murine orthotopic bladder xenografts. J Vis Exp 2014;e51123 [PubMed]
- Powles T, Eder JP, Fine GD, et al. MPDL3280A (anti-PD-L1) treatment leads to clinical activity in metastatic bladder cancer. Nature 2014;515:558-62. [Crossref] [PubMed]
- Shultz LD, Brehm MA, Garcia-Martinez JV, et al. Humanized mice for immune system investigation: progress, promise and challenges. Nat Rev Immunol 2012;12:786-98. [Crossref] [PubMed]
- Ito R, Takahashi T, Katano I, et al. Current advances in humanized mouse models. Cell Mol Immunol 2012;9:208-14. [Crossref] [PubMed]
- Rongvaux A, Willinger T, Martinek J, et al. Development and function of human innate immune cells in a humanized mouse model. Nat Biotechnol 2014;32:364-72. [Crossref] [PubMed]
- Brehm MA, Shultz LD, Luban J, et al. Overcoming current limitations in humanized mouse research. J Infect Dis 2013;208:S125-30. [Crossref] [PubMed]
- Shamir ER, Ewald AJ. Three-dimensional organotypic culture: experimental models of mammalian biology and disease. Nat Rev Mol Cell Biol 2014;15:647-64. [Crossref] [PubMed]
- Sato T, Vries RG, Snippert HJ, et al. Single Lgr5 stem cells build crypt villus structures in vitro without a mesenchymal niche. Nature 2009;459:262-5. [Crossref] [PubMed]
- Sato T, Stange DE, Ferrante M, et al. Long-term expansion of epithelial organoids from human colon, adenoma, adenocarcinoma, and Barrett's epithelium. Gastroenterology 2011;141:1762-72. [Crossref] [PubMed]
- Barker N, Huch M, Kujala P, et al. Lgr5+ ve stem cells drive self-renewal in the stomach and build long-lived gastric units in vitro. Cell Stem Cell 2010;6:25-36. [Crossref] [PubMed]
- Huch M, Dorrell C, Boj SF, et al. In vitro expansion of single Lgr5+ liver stem cells induced by Wnt-driven regeneration. Nature 2013;494:247-50. [Crossref] [PubMed]
- Antonica F, Kasprzyk DF, Opitz R, et al. Generation of functional thyroid from embryonic stem cells. Nature 2012;491:66-71. [Crossref] [PubMed]
- Karthaus WR, Iaquinta PJ, Drost J, et al. Identification of multipotent luminal progenitor cells in human prostate organoid cultures. Cell 2014;159:163-75. [Crossref] [PubMed]
- Leighton J, Tchao R, Tencer KL. Organoid structure of normal rat bladder in unilaminar and bilaminar histophysiologic gradient culture: methods and observations. In Vitro 1984;20:183-97. [Crossref] [PubMed]
- Huygens A, Kamuhabwa AR, Roskams T, et al. Permeation of hypericin in spheroids composed of different grade transitional cell carcinoma cell lines and normal human urothelial cells. J Urol 2005;174:69-72. [Crossref] [PubMed]
- Fujiyama C, Jones A, Fuggle S, et al. Human bladder cancer invasion model using rat bladder in vitro and its use to test mechanisms and therapeutic inhibitors of invasion. Br J Cancer 2001;84:558. [Crossref] [PubMed]
- Nakamura K, Fujiyama C, Tokuda Y, et al. Bladder cancer cell implantation in reconstructed bladder in vitro: a model of tumour recurrence. BJU Int 2002;89:119-25. [Crossref] [PubMed]
- Smith YC, Grande KK, Rasmussen SB, et al. Novel three-dimensional organoid model for evaluation of the interaction of uropathogenic Escherichia coli with terminally differentiated human urothelial cells. Infect Immun 2006;74:750-7. [Crossref] [PubMed]
- Janssen DA, Geutjes PJ, Odenthal J, et al. A new, straightforward ex vivo organoid bladder mucosal model for preclinical research. J Urol 2013;190:341-9. [Crossref] [PubMed]
- Palmer S, Litvinova K, Dunaev A, et al. Changes in autofluorescence based organoid model of muscle invasive urinary bladder cancer. Biomed Opt Express 2016;7:1193-200. [Crossref] [PubMed]
- Fatehullah A, Tan SH, Barker N. Organoids as an in vitro model of human development and disease. Nat Cell Biol 2016;18:246-54. [Crossref] [PubMed]
- Inc. G. TECENTRIQ (atezolizumab) injection. Prescribing Information. 2016. Available online: https://www.accessdata.fda.gov/drugsatfda_docs/label/2016/761034s000lbl.pdf