Lipid transactions in cancer—the fat addiction and glycerol-3-phosphate acyltransferase action
Metabolomics in cancer—what lipids have got to do?
Cancer is a complex-multistep process comprising genetic, molecular and metabolic events. Cancer cells are highly proliferative and invasive, always associated with an upregulation in their “natural building blocks”—nucleic acids, proteins and lipids. Due to a high genetic burden and also because of increasing structural and functional demands, a perturbation arises in the levels of numerous metabolites in biochemical pathways related to these building blocks. The metabolic signatures in cancer have been documented to reach diagnostic accuracy, serving as potential biomarkers. Metabolomics profile was explored in a wide range of cancers so far, including ovarian cancer, oesophago-gastric cancer, colorectal cancer etc., and dysregulation was noted in the pathways of cellular respiration, carbohydrate, protein, nucleotide and lipid metabolism (1). Metabolome shift is pivotal to cancer progression and among the key metabolic pathways, glucose and glutamine metabolism were widely highlighted, and phospholipid-choline metabolism are among the recently dissected candidates. The most popular metabolic change in cancer is the “Warburg effect”, characterized by heightened anaerobic glycolysis (lactic acidosis), where ATP generation is significantly obliterated. “Warburg effect” was one of the preliminary observations in cancer, but years later deviation was reported in several other pathways.
Lipids were previously considered only as passive cell membrane components. Pertaining to the lipid metabolism, common alterations in cancer include enhanced fatty acid oxidation, abnormal levels of glycerolipids, sphingolipids and free fatty acids (FA) etc (1). This metabolic rewiring is particularly because of microenvironment stimuli like hypoxia and starvation notable in cancer. In normal healthy cells, FA have multifaceted fates, which encompass: energy storage [triacylglycerols (TAGs), lipid droplets], membrane formation [glycerophospholipids, cardiolipins (CLs) and sphingolipids], generation of signaling molecules [lysophosphatidic acid (LPA), phosphatidic acid (PA), eicosanoids] or oxidized to generate ATP, serving as fuel (2). The cellular FA pool is formed from, exogenous FA arising from blood stream, carried into cells via membrane FA binding proteins (CD36), FA transport proteins or FA translocase or through passive entry; “mono and polyunsaturated FA, cholesterol” can form CLs, sphingomyelins, TAGs, phosphoglycerols, prostaglandins and thromboxanes. FA in cancer is routed through exogenous and endogenous source (2). To support cellular proliferation and biosynthetic activities in cancer cell, an accelerated lipid metabolism is critical (3). In 1953, Medes et al. were the first to demonstrate lipid synthesis in tumor tissues (2) (Figure 1). Lipids metabolism is dominant in cancer, as FA are essential for the synthesis of cell membranes and signaling molecules, and evidence suggests that cell proliferation can be suppressed through reduction of FA availability to the cancerous cell. Moreover, cells with significant cancer potential like cancer stem cells and aggressive cancer cells were shown to have more lipid content, and elevated anabolic (lipogenic) and catabolic (lipolytic) switching. FA metabolism is a potential metabolic program in cancer, but for a long time was a missing target for metastatic cancer treatment. Recently several reports, including the extensive studies of Rosemarie Marchan (RM) group, have documented the critical role of lipids in cancer survival and progression, from membrane building and signaling perspective. Among the six hallmarks of cancer (4), the contribution of lipids is mainly towards limitless proliferation and invasiveness–metastasis (3). For the modulation of lipid synthesis glycerol-3-phosphate acyltransferase (GPAT) is an effective target.
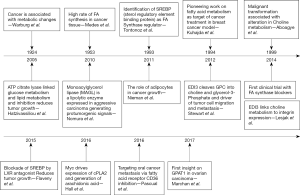
GPATs—their mundane roles in lipid metabolism
GPAT is composed of four isoforms that are critical to lipid homeostasis, and their deregulation forms the basis for pathology ranging from obesity and diabetes, to neurological disorders and cancer (5,6). Historically, esterification of glycerol-3-phosphate with a “long-chain acyl-CoA” was shown as the primary step in phospholipids synthesis.
Prospectively, Pullman group suggested that GPAT comprised of two isoforms, one in outer membrane of mitochondria and other in endoplasmic reticulum. The endoplasmic reticulum (microsomal) activity exhibited no preference for particular acyl-CoA species, whereas the mitochondrial activity preferred saturated acyl-CoAs (e.g., 16:0-CoA and 18:0-CoA) (7). As time passed, four genes were discovered encoding four separate GPAT isoenzymes. The GPAT1 and GPAT2 are mitochondrial isoforms, and GPAT3 and GPAT4 are endoplasmic reticulum isoforms (7). GPAT1 is resistant to sulfhydryl-modifying reagents like N-ethylmaleimide (NEM) inactivation, whereas other GPAT isoforms i.e., GPAT2, GPAT3 and GPAT4 are NEM sensitive (7). Much before, GPAT enzyme system was predicted to contain two set of isoforms, based on their kinetic profile, sensitivity to inhibitors and localization to the sub-cellular mitochondria and microsomes.
GPAT1 is an acyltransferase that
The knockout and overexpression studies clearly pointed at their putative role in development of hepatic steatosis (excess TAG in liver), insulin resistance, obesity, lactation, and spermatogenesis. In the recent times great emphasis has been put on transcriptional regulation of promoter of GPAT by “sterol regulatory element-binding transcription factor 1” (SREBP-1c) regulated by insulin (7). Ideally, optimal orchestration of GPAT system and other lipogenic genes [e.g., FA synthase (FASN)] is necessary for optimal lipogenesis. From animals (12) to humans, GPAT is a potential target to arrest the formation of downstream lipids that support cell membrane formation and downstream signaling molecules like LPA and PA that allow survival and proliferation of rapidly expanding cellular population in cancer (3). The GPAT enzymes have central role in lipid homeostasis and have been indicated in a large number of human cancers.
Linking endometrial differential 3 (EDI3) and downstream GPATs with cancers—how intimate is their relationship?
The role of EDI3 in cancer was first pointed by a team of gynecologists during an attempt to identify metastatic markers in endometrial carcinomas (13). The mechanistic studies were missing at that time, and later investigations confirmed it as a member of glycerophosphodiesterase enzyme family. The substrate of this enzyme and two cleavage products were identified around the same time. The challenge that remained was linking this enzymatic pathway to metastasis. Through lipid analysis Prof. Gerd Schmitz group identified that when EDI3 was manipulated the lipid profile (LPA and PA) altered significantly (13). These lipid mediators were already shown to activate signaling pathways, including migration, adhesion, and proliferation, which are essential to cancer progression. Experiments went on and EDI3 was shown in many models to be consistently linked to cellular migration.
Around 2012, RM group hypothesized that glycerophosphodiesterase EDI3 either directly generates the signaling molecules or provides the membrane anchors for downstream signaling factors (14,15). The EDI3 enzyme was identified to catalyze breakdown of glycerophosphocholine (GPC) to glycerol-3-phosphate and choline (14) (Figure 2). Choline is metabolized to phosphatidylcholine (PtdCho), a major lipid in plasma membrane and player in membrane signaling (14). The second product “glycerol 3 phosphate” (G3P) is a precursor to several lipids participating in signaling like LPA, PA, monoacyl-G3P, and diacylglycerol (DAG) (14). LPA has been established as a mitogen and motility factor but its intimate relationship to GPAT was then unknown. It also increases active protein kinase C (PKC) and enhances the migratory ability of tumor cells which signals through six G-proteins coupled receptors (6). Originally EDI3 was hypothesized to induce cell migration through the activation of PKC. In 2014, the same group identified a link between EDI3 and integrin signaling; they showed that EDI3 knockdown in breast and ovarian carcinoma cell line, led to “enrichment of genes in integrin mediated signaling”. Knockdown of EDI3 led to reduced expression of “integrin β1 receptor subunit”, a key integrin for cell attachment, leading to decreased cellular attachment and spreading accompanied by delayed formation of cellular protrusions (16). The tumors overexpressing EDI3 showed higher risk of metastasis and decreased survival, linking this protein to metastasis and survival (16).
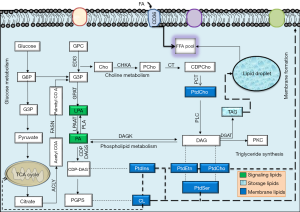
But putative downstream mediators of EDI3 were recently exposed by the RM group as being mediated via GPAT (17) (Figure 2). Among 13 cancer subtypes through bioinformatics approach, high ectopic GPAT2 expression has been identified by Baro group in a range of human cancers: melanoma, lung, prostate and breast cancer and relatively low expression was identified in renal, colorectal, hepatocellular, basal cell and hematological cancers (10). Before this, GPAT2 has been identified in multiple myelomas. Moreover the absence of GPAT2 produces induced apoptosis (9). GPAT2 knockdown and overexpression studies showed significant effect on cell proliferation, anoikis, migration and tumorigenicity, and staurosporine-induced apoptosis in several cell types (10). The increased expression of GPAT2 with a methyltransferase inhibitor suggested epigenetic regulation as its underlying mechanism. Overall, GPAT2 is strongly adherent to malignancy than glycerolipid synthesis, functionwise slightly different from its isoforms (10). The mechanism of activation of GPAT2 in spermatogenesis and its ectopic overexpression in cancer is through demethylation, i.e., epigenetic activation. Glycerophosphodiesterase EDI3 has been suggested to promote cell migration, adhesion and spreading, by the recent study of RM group (17). RM group targeted the GPAT1 along with choline kinase-α (CHKA), the enzymes that catabolize the products of EDI3-“G3P and choline” respectively, to determine which potential downstream pathways are precisely responsible for cellular migration (17). Their results clearly indicate that GPAT1 influences cell migration through “LPA signaling”. In the recent study, high GPAT1 expression was also observed in ovarian cancer patients with diminished survival. Also gene silencing experiments in ovarian cancer cell lines decreased cellular migration and growth of tumor xenografts (17). Favoring these observations, manipulating CHKA did not influence cell migration in the same set of cell lines. Based on these potential findings, RM group identified that GPAT1 influences intracellular LPA levels thereby promoting cell migration and tumor growth. Recently, LPA, the product of GPAT1, was also recently identified as essential to mitochondrial fusion, and GPAT1 mutants (C. elegans animal and Hela cell model) displayed mitochondrial fragmentation (18). Disturbed mitochondrial parameters like mitochondrial fusion rate has been linked to cancer; however the contribution of this mechanism to cellular migration was not exposed with respect to GPAT1 by RM group colleagues. Under normal conditions, mitochondrial morphology is governed by continuous fusion and fission events. Coleman group identified transgenic mice with GPAT1 deficiency to show reduced susceptibility to hepatocellular carcinoma from exposure to diethylnitrosamine and phenobarbital (7), adding weight to fundamental role of elevated GPAT1 to lipid synthesis in cancer. Recently through transcriptome derived datasets, a range of phospholipid breakdown products (including LPA) and eicosanoids were identified among a panel of mediators in ovarian tumor cells and tumor associated macrophages obtained through ascites sampling. Monitoring the levels of LPA for example may be helpful in early identification of relapse cases and tumor aggressiveness (prognostic markers?). It is of considerable interest that different LPA sps exert different biological effect and their characterization (pertained to FA-sn1 position) may be more helpful.
Choline metabolites, phosphocholine (PCho) and choline are universal to human cancers and have also been used to monitor increased tCho signal via 1H magnetic resonance spectroscopy and choline PET, allowing molecular imaging for early cancer detection (19). Metabolic products have been linked to cancer development and choline metabolism especially has to be considered as a potential cancer target.
Pharmacological inhibition of GPAT in cancer management
We appreciate the elaborate work of RM group who identified potential lipidomic pathways responsible for cancer metastasis. Dissecting such potential pathways/achilles heel in cancer is challenging and critical for drug design against enzymes catalyzing rate limiting steps. Uncontrolled proliferation and cellular migration are classic features in all human cancers, and their arrest is possible through blockage of key phospholipid enzymes like GPAT1. This may be possible by the antagonists of GPATeg: FSG67 (GPAT-1,-2 inhibitor), 2-(nonylsulfonamido) benzoic acid (15 g) (5,20). Based on available evidence design of small molecule inhibitors against GPAT may be effective for cancer therapeutics besides their roles in control of chronic diseases like obesity and type 2 diabetes. The same result would also be achieved by the blockage of type 1 LPA receptor (e.g., Ki16425) in cancer cells, which was already shown to reduce bony metastasis in animal models (12).
Acknowledgments
Funding: BM lab is supported by the Department of Biotechnology (DBT) and Science and Engineering Research Board, Department of Science and Technology (SERB-DST), India.
Footnote
Provenance and Peer Review: This article was commissioned and reviewed by the Section Editor Zheng Li (Department of Gynecologic Oncology, The Third Affiliated Hospital of Kunming Medical University, Kunming, China).
Conflicts of Interest: Both authors have completed the ICMJE uniform disclosure form (available at http://dx.doi.org/10.21037/tcr.2017.09.26). The authors have no conflicts of interest to declare.
Ethical Statement: The authors are accountable for all aspects of the work in ensuring that questions related to the accuracy or integrity of any part of the work are appropriately investigated and resolved.
Open Access Statement: This is an Open Access article distributed in accordance with the Creative Commons Attribution-NonCommercial-NoDerivs 4.0 International License (CC BY-NC-ND 4.0), which permits the non-commercial replication and distribution of the article with the strict proviso that no changes or edits are made and the original work is properly cited (including links to both the formal publication through the relevant DOI and the license). See: https://creativecommons.org/licenses/by-nc-nd/4.0/.
References
- Armitage EG, Southam AD. Monitoring cancer prognosis, diagnosis and treatment efficacy using metabolomics and lipidomics. Metabolomics 2016;12:146. [Crossref] [PubMed]
- Röhrig F, Schulze A. The multifaceted roles of fatty acid synthesis in cancer. Nat Rev Cancer 2016;16:732-49. [Crossref] [PubMed]
- Hanahan D, Weinberg RA. Hallmarks of cancer: the next generation. Cell 2011;144:646-74. [Crossref] [PubMed]
- Currie E, Schulze A, Zechner R, et al. Cellular fatty acid metabolism and cancer. Cell Metab 2013;18:153-61. [Crossref] [PubMed]
- Scott SA, Mathews TP, Ivanova PT, et al. Chemical modulation of glycerolipid signaling and metabolic pathways. Biochim Biophys Acta 2014;1841:1060-84. [Crossref] [PubMed]
- Yung YC, Stoddard NC, Mirendil H, et al. Lysophosphatidic Acid signaling in the nervous system. Neuron 2015;85:669-82. [Crossref] [PubMed]
- Ellis JM, Paul DS, Depetrillo MA, et al. Mice Deficient in Glycerol-3-Phosphate Acyltransferase-1 Have a Reduced Susceptibility to Liver Cancer. Toxicol Pathol 2012;40:513-21. [Crossref] [PubMed]
- Karlsson EA, Wang S, Shi Q, et al. Glycerol-3-phosphate acyltransferase 1 is essential for the immune response to infection with coxsackievirus B3 in mice. J Nutr 2009;139:779-83. [Crossref] [PubMed]
- Garcia-Fabiani MB, Montanaro MA, Stringa P, et al. Glycerol-3-phosphate acyltransferase 2 is essential for normal spermatogenesis. Biochem J 2017;474:3093-107. [Crossref] [PubMed]
- Pellon-Maison M, Montanaro MA, Lacunza E, et al. Glycerol-3-phosphate acyltranferase-2 behaves as a cancer testis gene and promotes growth and tumorigenicity of the breast cancer MDA-MB-231 cell line. PLoS One 2014;9:e100896 [Crossref] [PubMed]
- Cao J, Perez S, Goodwin B, et al. Mice deleted for GPAT3 have reduced GPAT activity in white adipose tissue and altered energy and cholesterol homeostasis in diet-induced obesity. Am J Physiol Endocrinol Metab 2014;306:E1176-87. [Crossref] [PubMed]
- Boucharaba A, Serre CM, Guglielmi J, et al. The type 1 lysophosphatidic acid receptor is a target for therapy in bone metastases. Proc Natl Acad Sci U S A 2006;103:9643-8. [Crossref] [PubMed]
- Marchan R, Stewart JD, Lesjak M. EDI3, a key enzyme of choline metabolism controls tumour cell migration. EXCLI J 2012;11:260-2. [PubMed]
- Marchan R, Lesjak MS, Stewart JD, et al. Choline-releasing glycerophosphodiesterase EDI3 links the tumor metabolome to signaling network activities. Cell Cycle 2012;11:4499-506. [Crossref] [PubMed]
- Stewart JD, Marchan R, Lesjak MS, et al. Choline-releasing glycerophosphodiesterase EDI3 drives tumor cell migration and metastasis. Proc Natl Acad Sci U S A 2012;109:8155-60. [Crossref] [PubMed]
- Lesjak MS, Marchan R, Stewart JD. EDI3 links choline metabolism to integrin expression, cell adhesion and spreading. Cell Adh Migr 2014;8:499-508. [Crossref] [PubMed]
- Marchan R, Büttner B, Lambert J, et al. Glycerol-3-phosphate acyltransferase 1 promotes tumor cell migration and poor survival in ovarian carcinoma. Cancer Res 2017;77:4589-601. [Crossref] [PubMed]
- Ohba Y, Sakuragi T, Kage-Nakadai E, et al. Mitochondria-type GPAT is required for mitochondrial fusion. EMBO J 2013;32:1265-79. [Crossref] [PubMed]
- Glunde K, Penet MF, Jiang L, et al. Choline metabolism-based molecular diagnosis of cancer: an update. Expert Rev Mol Diagn 2015;15:735-47. [PubMed]
- Wydysh EA, Medghalchi SM, Vadlamudi A, et al. Design and synthesis of small molecule glycerol 3-phosphate acyltransferase inhibitors. J Med Chem 2009;52:3317-27. [Crossref] [PubMed]